Volume 12 - Year 2024 - Pages 60-65
DOI: 10.11159/ijepr.2024.008
An Approach to Alternative Energy Management of Hydropower for Green Hydrogen Production
Selisa R. Andrus1, Terry L. Alford1*
1Arizona State University, School for Engineering of Matter, Transport and Energy
Tempe, Arizona United States 85287
*Corresponding Author: TA@asu.edu
Abstract - The potential for green hydrogen from resource management presents an unparalleled opportunity to explore multiple-use hydropower systems for green hydrogen production. This study demonstrates river systems with inadequate reservoir storage capacities (e.g., run-of-river projects) can be feasible for green hydrogen generation via electrolysis. The research revealed surplus hydroelectric energy from a controlled river system is most prevalent during March through July, wherein these months are the driving factors for hydrogen generation possibilities. A semi-monthly deterministic model was used to identify excess energy capabilities over eight decades of water years. Outcomes reveal the total month-to-month medium hydrogen generation at run-of-river projects varies at 2 - 8 ×106 kilograms of H2. This research indicates hydrogen generation from excess water from hydropower has the potential to be a feasible fuel alternative in the heavy-duty transport industries.
Keywords: Green Hydrogen Production, Hydropower, Power-to-Hydrogen, Run-of-River Systems
.© Copyright 2024 Authors - This is an Open Access article published under the Creative Commons Attribution License terms. Unrestricted use, distribution, and reproduction in any medium are permitted, provided the original work is properly cited.
Date Received: 2023-01-22
Date Revised: 2024-07-18
Date Accepted: 2024-08-16
Date Published: 2024-12-12
1. Introduction
Reducing greenhouse gas emissions from the transportation sector is a significant challenge. Heavy-duty transportation, such as trucks, buses, and airplanes, relies heavily on fossil fuels and is challenging to electrify with current technologies due to the high energy requirements and limited range [1]. As a result, reducing emissions from the heavy-duty transportation sector will likely require a combination of different strategies, including alternative fuels and efficiency improvements. One alternative resource that shows promise for heavy-duty transportation is hydrogen fuel cells [2]. While this technology is still in the early stages of development and faces significant challenges, it holds promise for the long-term decarbonization of the heavy-duty transportation sector.
However, challenges are associated with the widespread adoption of hydrogen as an energy carrier, including the high cost of production, storage, and distribution and the need for significant infrastructure investments. The U.S. Infrastructure Investment and Jobs Act, which was recently passed, includes substantial funding for clean energy infrastructure and transportation, including support for developing clean hydrogen hubs [3, 4]. This legislation can provide a pathway for federal and state governments to accelerate the adoption of low-carbon transportation technologies and reduce emissions from the transportation sector.
Hydrogen can potentially play a significant role in decarbonizing the transportation sector. Most hydrogen produced in the United States is currently produced through steam methane reforming [5]. The deployment of clean alternatives such as electrolysis is rapidly increasing; however, it is currently not economical [6]. Using renewable energy sources (RESs) such as hydropower, wind, and solar for green hydrogen production can significantly reduce carbon dioxide (CO2) emissions and increase energy security [7].
A growing body of research explores theoretical scenarios in which curtailed energy from solar and wind is realized for various applications. These studies suggest significant potential for green hydrogen production to play a vital role in decarbonizing the transportation sector and other industries while augmenting the integration of RESs. However, solar and wind variability and non-dispatchable nature present challenges related to cost, regulatory, and operational constraints associated with green hydrogen production. Furthermore, the practical implementation of these solutions requires continued research and development and significant investment in infrastructure and deployment.
On the contrary, using excess energy from hydropower plants offers a unique approach to producing green hydrogen. Although considered a RES, hydropower has an advantage over solar and wind due to its dispatchable capability. There are two main classifications of hydropower plants – storage and run-of-river systems [8, 9]. Storage systems comprise reservoirs that enable water accumulation during inflow peaks. This stored water can be released during periods of low inflows to meet flood control, fish passage, environmental quality, and electricity needs. Run-of-river systems typically have limited storage capacity or short residence time of water runoff and electricity generation depends on timing and the local volume of river flow.
Limited studies have investigated the utilization of excess water accumulation from run-of-river hydropower plants for green hydrogen production [10, 11]. These case studies demonstrated feasibility of green hydrogen production in Slovenian run-of-river hydropower plants. Key results from these studies concluded excess hydropower from run-of-river systems used for hydrogen cogeneration is economically viable.
2. Case Study
This paper investigates the potential for hydrogen production from excess hydropower generation from a controlled water system in the Pacific Northwest region of the United States. The high annual runoff in the Columbia River Basin relative to its storage capacity has several implications for water management in the Pacific Northwest region [12]. One of the primary challenges is balancing the need for hydropower generation with other competing demands for water, such as irrigation, municipal water supply, and fish and wildlife habitats. Water shortages can occur in dry years or during extended droughts, impacting all these sectors. Additionally, climate change is expected to exacerbate these challenges by altering precipitation patterns and increasing the frequency and severity of extreme weather events.
The Columbia River Basin's unique hydrology presents challenges and opportunities for water management. The available reservoir storage in the basin is on the order of 7 ×1010 m3/yr; whereas, the average annual runoff from the basin is over 3 ×1011 m3 per year [13]. By implementing innovative and adaptive strategies, stakeholders can work together to ensure that water resources are managed sustainably and equitably in the face of ongoing hydrologic variability. The Federal Columbia River Power System (FCRPS) plays a critical role in meeting the Pacific Northwest region's energy demand and water needs [14]. The FCRPS operates under the auspices of the federal government in coordination with state and local partners to achieve a range of congressionally authorized purposes.
Hydropower production is one of the primary purposes of the FCRPS. The dams and reservoirs in the system generate electricity that is sold to utilities throughout the region. The hydroelectric power produced by the system is a key component of the region's energy mix, providing a reliable and low-cost source of electricity that is also carbon-free. During times of water surplus, particularly in the spring and summer runoff periods, hydropower surpluses can displace gas-fired and coal-fired power generation at a relatively low cost with zero carbon emissions. However, there is a limit to how much thermal generation can be displaced, and once that limit is reached, oversupply can result in the curtailment of clean energy. This dynamic is illustrated in Figure 2, wherein forecasted hydropower and non-hydropower RESs peak in the months of April through July.
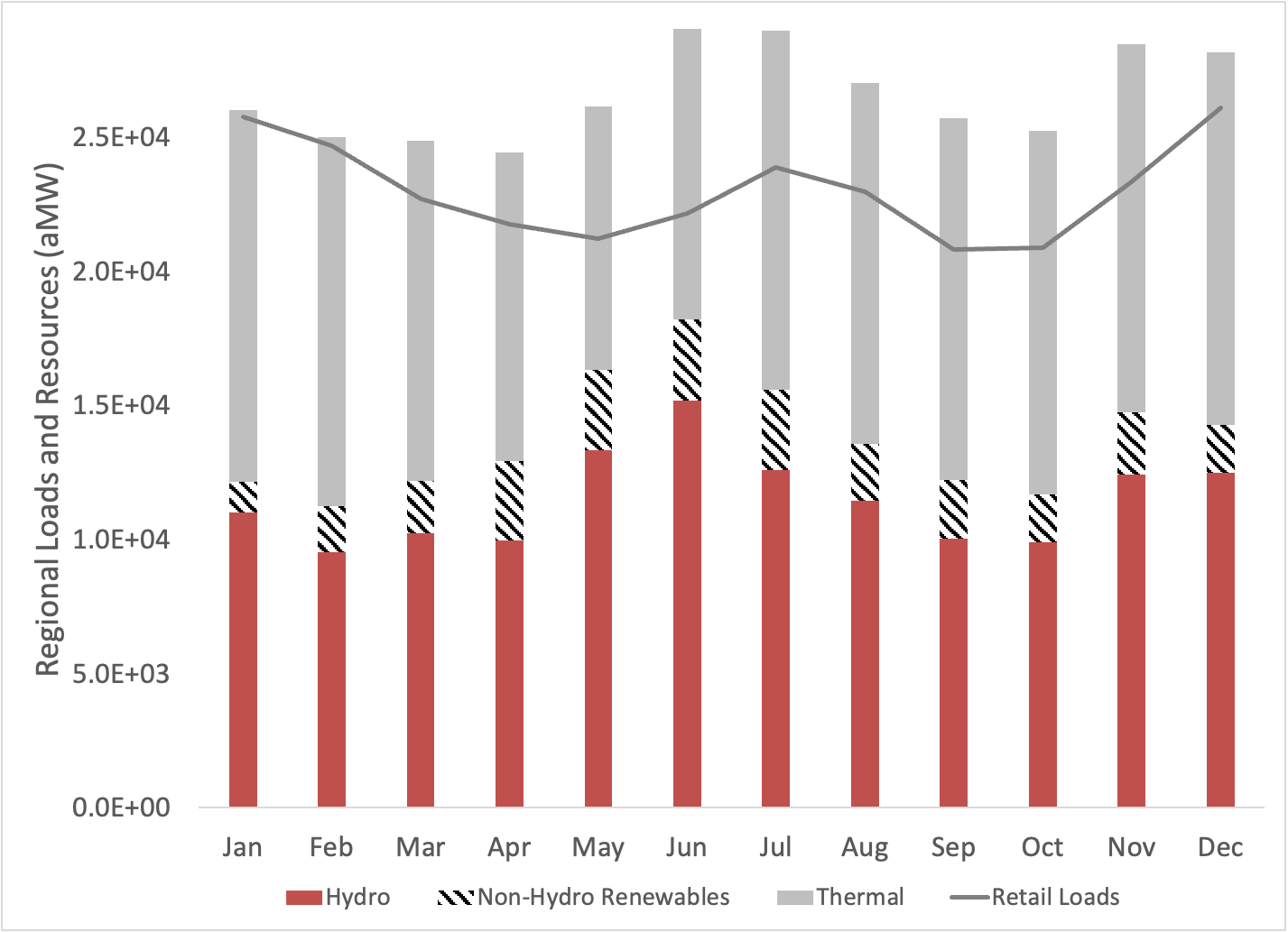
The Bonneville Power Administration (BPA) uses a hydrologic simulation and deterministic model called HydroSystem Simulator (HYDSIM) for power modeling, incorporating flood risk management, spill regimes, and other operating criteria and constraints [15]. This model calculates results in strict accordance with its rule logic and has been historically used by BPA to complete studies of system-wide hydropower operations. Furthermore, the model operates on a quasi-monthly timestep that simulates the hydropower system's reservoir, powerhouse, and dam operations over a period.
2. 1. Methodology
The HYDSIM model in this study applies the 2010 Level Modified Flows for water year sequences 1929 through 2008 as input and is run in continuous mode [15]. In continuous mode, the same load and resource parameters are applied to all water years, and the ending elevations for each historical water year become the starting elevations for the next water year. This modeling approach allows BPA to evaluate the performance of the hydropower system under various scenarios and conditions, including changes in water availability and climate.
The rate case is a biennial study conducted by BPA which focuses on modeling power operations to ensure that projected revenue from the electricity the agency sells will cover the expenses it incurs [16]. Resulting data sets from the BP-22 rate case provide information on project outflows, reservoir elevations, reservoir contents, spillway flows, and power generation, which can be used to calculate overgen spill at hydroelectric projects. Overgen spill is the excess water that cannot be used for power generation and is spilled over the dam. By using historical streamflow data that accounts for current irrigation depletions and the effects of river regulation, the study can provide insights into how the FCRPS can be operated most efficiently and effectively possible while minimizing environmental impacts.
Defined HOVK tables are used in the HYDSIM model to calculate the power generation from hydroelectric projects. HOVK measures the energy conversion efficiency of the turbines, which varies with the head of water. The head is the elevation difference between the reservoir's water surface and the turbine's outlet. The factor k is a unitless coefficient that represents the turbine efficiency, which is a function of the design of the turbine and generator.
The HOVK tables provide a lookup table of h/k values for different values of head, allowing the HYDSIM model to interpolate values for each period. Once the HOVK value is obtained, the model can calculate the expected power generation for a given flow rate. This information is then used to calculate overgen spill at each hydroelectric project. The energy (in MW) by overgen spill flow through generation/turbine produced is given by:
Total Overgen spill (MW) = Σ (kWm-3s-1) Qspill t
where Σ is the summation of kW produced per second, Qspill is the spill rate in cubic meters per second, and t is time in seconds.
Spilled hydropower can produce the amount of power required needed by an electrolyzer to produce H2 is given by [17]:
PH2 = 3600 × Qspill × (HHV / VH2)
where PH2 is the potential hydrogen production in cubic meters per hour, HHV is the higher heating value of hydrogen in megajoules per cubic meter, and VH2 is the volume of hydrogen produced by the electrolysis of one cubic meter of water, which is approximately 0.0111 cubic meters at standard temperature and pressure. The factor of 3600 is used to convert the spill rate from cubic meters per second to cubic meters per hour.
3. Results
The FCRPS projects examined in detail for this study were two storage (Grand Coulee and Dworshak) and eight run-of-river projects (Bonneville, Chief Joseph, Ice Harbor, John Day, Little Goose, Lower Monumental, McNary, and The Dalles). Operational properties of these hydropower systems are described elsewhere in the literature [16]. The passage suggests wasted hydroelectric energy from these projects can be converted to hydrogen, which can then be used in fuel cell systems to produce electricity. The study found the production of hydrogen from spilled hydropower energy is most prevalent during the spring through summer runoff periods when there is an observed surplus of energy. The results shown in Figure 2 also suggest run-of-river hydroelectric projects are more viable than storage projects for producing hydrogen from overgen spill.
The large variation (σ = 2.3 x 106) in total hydrogen production for run-of-river versus storage projects suggests water availability and operational constraints play a significant role in electricity generation. Run-of-river projects provide a continuous supply of electricity; whereas, storage projects provide not only base load but offer operational flexibility.
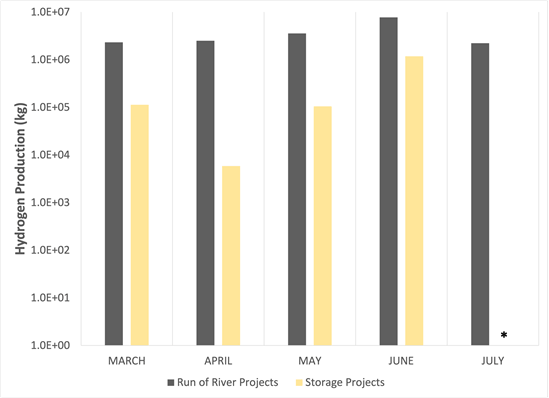
This study relied on the HYDSIM model, which predicts potential hydrogen production from each hydroelectric project over an 80-year water record. However, the model does not capture the fluctuation in water flow accurately as a short-term model (e.g., daily or hourly). The lack of granularity in the data is a significant limitation of the study; but, it also presents an opportunity for future work. For example, resource adequacy tool, GENESYS (Generation Evaluation System), a constrained economic dispatch model that employs Monte Carlo sampling to assess the effects of uncertainty in demand, stream flows, solar/wind generation, and forced outages can be utilized to assess hydrogen production from overgen spill on a planning basis.
The transportation sector, in general, is the largest contributor to greenhouse gas emissions in the United States, accounting for about 28% of total emissions in 2019. Therefore, reducing emissions from heavy-duty vehicles is critical to addressing climate change and improving air quality. The equivalent CO2 emissions avoided by select run-of-river projects analyzed in this study are shown in Figure 3. The figure suggests the month of June is the most prevalent period for emissions reduction due to the surplus of hydropower and the inability to store river runoff.
In comparison, storage projects such as Grand Coulee and Dworshak analyzed in this study (Figure 4) did not display significant hydrogen production. They did reduce CO2 emissions during the months of April and July due to the need for these projects to store water for flood risk management purposes.
The transportation sectors in Washington State and Oregon accounted for 51 and 55 percent, respectively, of total energy-related CO2 emissions [18]. An estimation of the encouraging environmental impact of renewable hydrogen for heavy-duty transportation use in the Northwest region was addressed elsewhere and determined the total amount of CO2 emissions associated with diesel consumption for transport in the states of Oregon and Washington was approximately 1.5×106 metric tons in the year 2020. Eliminating the total CO2 emitted by diesel combustion in Oregon and Washington in 2020 would cost between 1.4 - 3.5 billion U.S. dollars.
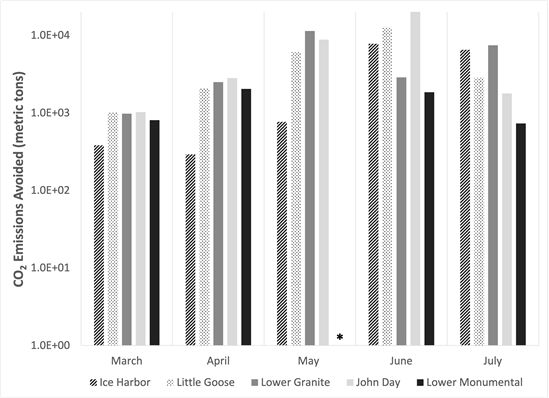
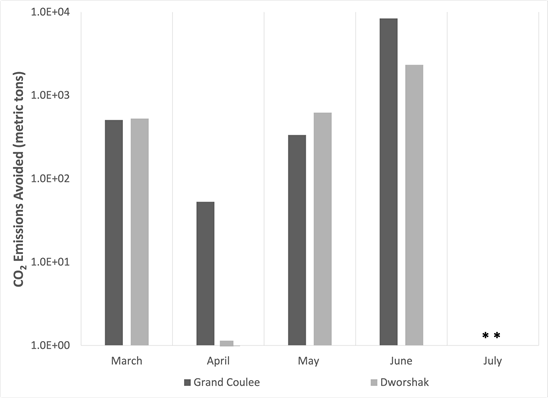
Although a direct comparison of hydrogen and diesel-fueled heavy-duty vehicles can be part of assessing the value of hydrogen fuel for transport applications in the Pacific Northwest, it may not provide a complete picture. A more comprehensive analysis can consider various factors, including the availability and cost of hydrogen infrastructure, the efficiency and emissions of hydrogen production and distribution, and the overall benefits and costs of transitioning to hydrogen fuel in the transport sector.
Several studies have assessed the production cost of grid-based electrolytic hydrogen across the United States and concluded that hydrogen could be cost-effective for current energy and transportation systems, especially when produced from excess renewable energy. The production cost of grid-based electrolytic hydrogen depends on several factors, including the cost of electricity, the efficiency of the electrolysis process, and the capital and operating costs of the equipment.
A report shares that the production cost of grid-based electrolytic hydrogen that hydrogen can be cost-valuable for future transportation sectors, given that electrolysis-based hydrogen production costs range from 2.6 to 12.3 U.S. dollars per kg of H2 [19]. The cost of the 2 ×107 kg of H2 that can be produced via run-of-river projects along the FCRPS would cost an average of 137 million U.S. dollars. The monetary value of these emissions savings can be estimated based on the cost of capturing and storing CO2 through carbon capture and storage (CCS) technology. The cost of CCS varies widely depending on the specific technology used, but it can range from 54 - 109 U.S. dollars per metric ton of CO2 captured and stored [20]. Based on this range, the average emissions savings from generating hydrogen via excess run-of-river hydropower through electrolysis is 28 million U.S. dollars per year.
Conclusion
Using excess hydropower from run-of-river plants to generate hydrogen through electrolysis can help increase the power grid's overall efficiency. Excess hydropower can produce hydrogen during periods of low demand, which can help balance the power grid and reduce the need for energy storage. Furthermore, using hydrogen as a fuel can provide a range of benefits, including increased efficiency, reduced emissions, and improved energy security. Hydrogen can be used to power fuel cells that can generate high efficiency and zero emissions and can also be used as fuel for transportation, heating, and industrial processes. Overall, using excess hydropower from run-of-river systems to generate hydrogen through electrolysis is a promising development that can help increase the power grid's flexibility and efficiency while reducing emissions and improving energy security. In a case study of the FCRPS where variable water conditions over an 80-year period were considered, the projected hydrogen production from eight hydroelectric run-of-river plants is approximately 2×107 kg per year. This produced hydrogen is equivalent to 2×105 metric tons of CO2 emissions avoided and an average savings of 28 million U.S. dollars for CCS.
References
[1] Anselma, P.G., Belingardi, G.: Fuel cell electrified propulsion systems for long-haul heavy-duty trucks: present and future cost-oriented sizing. Appl Energy. 321, 119354 (2022) View Article
[2] Camacho, M. de las N., Jurburg, D., Tanco, M.: Hydrogen fuel cell heavy-duty trucks: Review of main research topics. Int J Hydrogen Energy. 47, 29505-29525 (2022) View Article
[3] Zeitler, E.: Opportunities for energy and climate sectors in the infrastructure investment and jobs act. Climate and energy. 38, 10-15 (2022) View Article
[4] Offutt, M.C.: Hydrogen hubs and demonstrating the hydrogen energy value chain, (2022)
[5] Navarro, M. V., Plou, J., López, J.M., Grasa, G., Murillo, R.: Effect of oxidation-reduction cycles on steam-methane reforming kinetics over a nickel-based catalyst. Int J Hydrogen Energy. 44, 12617-12627 (2019) View Article
[6] Pandiyan, A., Uthayakumar, A., Subrayan, R., Cha, S.W., Moorthy, S.B.K.: Review of solid oxide electrolysis cells: a clean energy strategy for hydrogen generation. Nanomaterials and energy. 8, 2-22 (2019) View Article
[7] Amado, N.B., Pelegia, E.D.B., Sauer, I.L.: Capacity value from wind and solar sources in systems with variable dispatchable capacity - An application in the Brazilian hydrothermal system. Energies (Basel). 14, 3196 (2021) View Article
[8] Anderson, D., Moggridge, H., Warren, P., Shucksmith, J.: The impacts of "run-of-river" hydropower on the physical and ecological condition of rivers. Water and environment journal: WEJ. 29, 268-276 (2015). View Article
[9] U.S. Department of Energy - Water Power Technologies Office: Types of Hydropower Plants, View Article
[10] Jovan, D.J., Dolanc, G., Pregelj, B.: Cogeneration of green hydrogen in a cascade hydropower plant. Energy Conversion and Management: X. 10, 100081 (2021). View Article
[11] Jovan, D.J., Dolanc, G., Pregelj, B.: Utilization of excess water accumulation for green hydrogen production in a run-of-river hydropower plant. Renew Energy. 195, 780-794 (2022). View Article
[12] Rupp, D.E., Chegwidden, O.S., Nijssen, B., Clark, M.P.: Changing River Network Synchrony Modulates Projected Increases in High Flows. Water Resour Res. 57, (2021) View Article
[13] Hyde, J.M.: Columbia River Treaty Past and Future. In: Proceedings of HydroVision International Conference. pp. 1-25. M. Barnes, PennWell Corporation, Charlotte, VA (2010) View Article
[15] Johnson, A., Racht, P., Wallace, S.: Hydro Modeling Update for the Columbia River System, (2021) View Article
[16] Columbia River System operations final environmental impact statement. U.S. Army Corps of Engineers-Northwestern Division, Portland, Or (2020)
[17] Andrus, S.R., Diffely, R.J., Alford, T.L.: Theoretical analysis of green hydrogen from hydropower: A case study of the Northwest Columbia River system. Int J Hydrogen Energy. 48, 7993-8001 (2023) View Article
[18] Lombardi, S., Tribioli, L., Guandalini, G., Iora, P.: Energy performance and well-to-wheel analysis of different powertrain solutions for freight transportation. Int J Hydrogen Energy. 45, 12535-12554 (2020) View Article
[19] Guerra, O.J., Eichman, J., Kurtz, J., Hodge, B.M.: Cost Competitiveness of Electrolytic Hydrogen. Joule. 3, 2425-2443 (2019) View Article
[20] Schmelz, W.J., Hochman, G., Miller, K.G.: Total cost of carbon capture and storage implemented at a regional scale: northeastern and midwestern United States. Interface Focus. 10, 20190065-20190065 (2020) View Article