Volume 8 - Year 2020 - Pages 23-29
DOI: 10.11159/ijepr.2020.003
Physico-Chemical and Ecotoxicological Characterizations of Suburban Soils
Dávid Mónok1, Levente Kardos1, Sándor Attila Pabar1, Zsolt Kotroczó1
1Department Department of Agro-Environmental Studies, Institute of Environmental Science, Szent István University
29-43 Villányi út, Budapest, Hungary, H-1118
Monok.David@hallgato.szie.hu
Abstract - The study aimed to evaluate the quality of some suburban soils in Budapest (Hungary) by integrating different physico-chemical and ecotoxicological approaches. Soils were sampled from six different sites and characterized for main soil properties (pH, CaCO3, humus, and water-soluble salt contents) and total-extractable metal concentrations (Cd, Co, Cr, Cu, Ni, Pb, Zn). Ecotoxicological characterization was carried out using test species from different taxonomic groups: Azomonas agilis and Pseudomonas fluorescens (bacteria), Sinapis alba, and Lactuca sativa (plants), Folsomia candida, and Eisenia fetida (invertebrates). All ecotoxicological tests performed showed some degree of toxicity due to contact with certain soil samples, however, the test organisms showed different sensitivities. The results showed that the dehydrogenase activity of P. fluorescens, the germination rate of the studied plants, and the reproduction rate of the invertebrates were the most sensitive toxicological endpoints. Based on our correlation analysis, the outcome of ecotoxicological tests was influenced by the humus content and heavy metal concentration of the soil.
Keywords: Ecotoxicology, Ecotoxicological tests, Heavy metals, Suburban soils, Urban soils
© Copyright 2020 Authors - This is an Open Access article published under the Creative Commons Attribution License terms. Unrestricted use, distribution, and reproduction in any medium are permitted, provided the original work is properly cited.
Date Received: 2020-11-07
Date Accepted: 2020-11-12
Date Published: 2020-12-23
1. Introduction
According to various previous studies, human activities have a very strong influence on the soil characteristics of urban areas [1]–[4]. Urban soils generally have high bulk densities, high pH, and carbonate content, and often contain certain pollutants that pose potential risks to ecosystem members [2]-[4]. One of the main pollutants in these soils is heavy metals, which can be accumulated in topsoil at relatively high concentrations [2], [5], [6]. As anthropogenic pollution of heavy metals is not expected to decrease globally, urban soils may become major sources of secondary metal pollution [7].
In recent decades, many studies have been conducted to assess soil quality in different cities. Most of them focus on soils that are directly affected by human activity (e.g. soils in industrial or roadside areas), or on soils, which come easily in contact with humans, especially children’s (e.g. soils in parks or playgrounds) [5], [8], [9]. However, there are only a few examples of studying suburban soils in the literature, so little data are available on the soil characteristics of these areas. Nevertheless, the study of these soils is also important, as they bring fundamental benefits to urban residents (e.g., air purification, water, and climate regulation) and provide habitat for terrestrial communities [10], [11].
Although traditional physico-chemical test methods are suitable for assessing soil quality, supplementing them with ecotoxicological tests can provide an even more accurate picture of the condition of soils [12]-[15]. These tests are primarily suitable for estimating the potential risks (especially toxicity) of various effects on soil and pollution [12], [15]. For proper assessment of soil quality, the use of several taxonomically distinct test species that play different roles in the soil ecosystem is recommended during the ecotoxicological tests [16], [17].
The main objective of our study was to assess the soil quality and toxicity of soils originated from various suburban green sites. To achieve this, we combined traditional physico-chemical methods and ecotoxicological tests. Another objective was to search for correlations between the results in order to identify which soil characteristics influence the outcome of ecotoxicological tests.
2. Material and methods
2.1. Study area
The study was conducted in the eastern part of Budapest (the capital of Hungary), which is located in Central Europe. The climate of the area is (humid) continental and the soils here are generally sandy soils. Budapest has been inhabited since the Roman age, and it has also a long industrial history. Due to the long-term human activities, the natural state of the soil is likely to have changed significantly. The city has an area of 525 km2, however, only about 16 % of them are green areas, which are mainly located in the suburbs. Due to this relatively low rate, it is very important to preserve the green sites in good condition, which is not possible without monitoring suburban soils.
2.2. Study sites and soil sampling
The study was carried out at six sites, which were covered with grass vegetation (Figure 1.). All of them was park site or other recreational green areas. In each site, four composite samples were collected by mixing 10 subsamples taken randomly from the study site. All samples were originated from the upper layer of soil (0-20 cm).
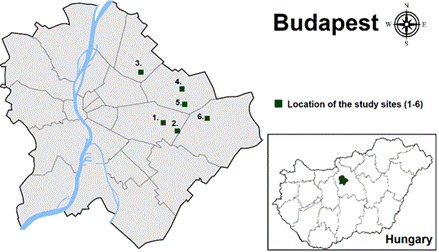
2.3. Physico-chemical methods
All soil samples were homogenized, air dried, and sieved through a 2 mm diameter sieve before the examinations. Soil textures were identified by particle size distribution with a hydrometer. Soil pH was measured with a pH meter in an H2O solution (1:2.5 soil/solution ratio), while the total salt (TS) contents were determined by a conductometer in another H2O solution (1:5 soil/solution ratio). The humus (H ty) contents were determined according to the Tyurin method, and the calcium carbonate (CaCO3) contents were measured with a Scheibler calcimeter. Total-extractable metal (Cd, Co, Cu, Cr, Ni, Pb, Zn) concentrations were recorded by atomic absorption spectrometry (AAS) after HNO3+H2O2 digestion.
2.4. Ecotoxicological methods
Six different test species were used during the ecotoxicological characterization of the soil samples: Azomonas agilis and Pseudomonas fluorescens (bacteria), Sinapis alba, andLactuca sativa (plants), Folsomia candida, and Eisenia fetida (soil invertebrates). The two bacterial tests were performed using the methods of previous studies [12], [14]. Plant and invertebrate tests were based on various OECD guidelines [18], [19], [20]. For these tests standard OECD soil (consisting of 70 % quartz sand, 20 % kaolinite clay, and 10 % sphagnum moss) was applied as control soil. The main features of the ecotoxicological tests are shown in Table 1.
Table 1. Main features of the applied ecotoxicological tests.
Species | Endpoints | Time | Interpretation |
A. agilis | dehydrogenase activity (DHA) | 3 days | IC50 value* |
P. fluorescens | |||
S. alba | germination rate, root elongation | 5 days | Inhibition (% of control) |
L. sativa | |||
F. candida | survival rate, reproduction rate | 4 weeks | |
E. fetida | 8 weeks |
Note: *soil dose resulting in a 50 % inhibition effect of bacterial dehydrogenase enzyme activity (DHA)
2.5. Statistical analyses of the results
Statistical analyses were performed using SPSS software. Kruskal-Wallis test (p < 0.05) was used to compare the results obtained from different sites. Spearman’s correlation (p < 0.05) was used to determine if there was a correlation between physico-chemical and ecotoxicological data.
3. Results and discussion
3.1. Results of physico-chemical characterization
The texture of the soils was clay loam or sandy clay loam, except for Site 6, where it was clay. The other physico-chemical parameters are shown in Table 2. Soil pH was close to neutral at all sites, while TS contents were between 0.06 and 0.08 %, which values are relatively low. At sites 4 and 6, the amount of Hty and CaCO3 in the soil was significantly higher than at some other sites. In general, the lowest heavy metal concentrations (excluding Cu and Zn) were observed at site 1, while the highest was observed at sites 3, 4, and 5. Cr concentrations at sites 3, 4, and 5 were significantly higher than at the other sites. And the Pb concentration was also significantly higher but only at site 3. Compared to previous studies, the concentrations of Cd, Cr, and Pb are relatively high in our soil samples [5], [6].
Table 2. Results of physico-chemical characterization of soil samples.
Site number | ||||||
1 | 2 | 3 | 4 | 5 | 6 | |
pH |
7.2
±0.1 AB |
7.1
±0.4 AB |
7.2
±0.2 AB |
7.0
±0.1 B |
6.7
±0.2 A |
7.5
±0.1 B |
TS*
(%) |
0.06
±0.01 A |
0.06
±0.01 A |
0.08
±0.01 A |
0.07
±0.01 A |
0.04
±0.01 A |
0.08
±0.01 A |
Hty+ (%) |
3.3
±0.5 A |
2.4
±0.3 A |
2.7
±0.5 A |
6.0
±0.4 B |
4.4
±0.5 AB |
6.4
±0.5 B |
CaCO3 (%) |
0.5
±0.5 A |
5.2
±1.2 AB |
5.1
±1.0 AB |
7.5
±0.6 B |
0.8
±0.6 A |
8.7
±1.1 B |
Cd (mg kg-1) |
1.5
±0.3 A |
1.6
±0.4 AB |
2.7
±0.3 B |
2.7
±0.2 B |
2.3
±0.3 AB |
1.9
±0.3 AB |
Co (mg kg-1) |
7.7
±0.9 A |
9.2
±0.8 AB |
14.6
±1.2 AB |
14.1
±0.4 AB |
14.5
±0.2 B |
8.7
±1.1 AB |
Cr (mg kg-1) |
93.1
±6.1 AB |
111.9
±28.9 B |
220.9
±19.4 C |
231.2
±36.4 C |
248.9
±11.7 C |
63.7
±13.9 A |
Cu (mg kg-1) |
38.6
±4.6 A |
29.0
±6.9 AB |
29.8
±1.8 B |
31.6
±1.6 AB |
29.9
±8.0 AB |
37.4
±3.0 AB |
Ni (mg kg-1) |
22.9
±1.8 A |
20.4
±3.0 A |
31.3
±1.9 AB |
37.7
±3.0 B |
32.2
±2.8 AB |
37.4
±2.5 B |
Pb (mg kg-1) |
173.0
±12.8 A |
198.9
±39.4 AB |
292.8
±31.5 C |
210.0
±29.2 AB |
220.7
±18.7 B |
210.0
±15.3 AB |
Zn (mg kg-1) |
56.1
±13.1 A |
39.6
±5.7 AB |
44.8
±6.7 AB |
45.6
±5.9 AB |
33.0
±2.7 B |
48.2
±4.1 A |
Data are shown as means
± sd (n=4). Notes: *Total salt content, +
Humus content.
Different letters indicate significant differences between sites
(Kruskal-Wallis test, p < 0.05).
3.2. Results of ecotoxicological characterization
The results of the bacterial tests are shown in Figure 2. All samples were quite toxic to the test bacteria, even less than 1.5 g of soil inhibited the bacterial dehydrogenase enzyme activity by 50 %. Lower IC50 values were observed at sites 1, 2, and 3, however, there was no significant difference between sites according to the statistical analysis.
Among the two tests, the P. fluorescens test was found to be more sensitive than the A. agilis test, because then a lower soil dose caused the same inhibitory effect.
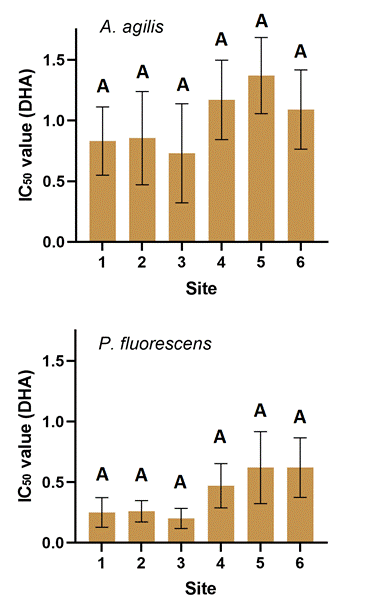
Results of plant bioassays showed that soils from Site 1, 2, and 3 were also highly toxic to the germination of the test plants (Figure 3.). These soils reduced the germination rate of the plants by about 50 % compared to the control. In contrast, the sample from site 5 was significantly less toxic to plants, decreasing plant germination by only about 5 %. The sample from sites 4 and 6 reduced this parameter by about 30 %.
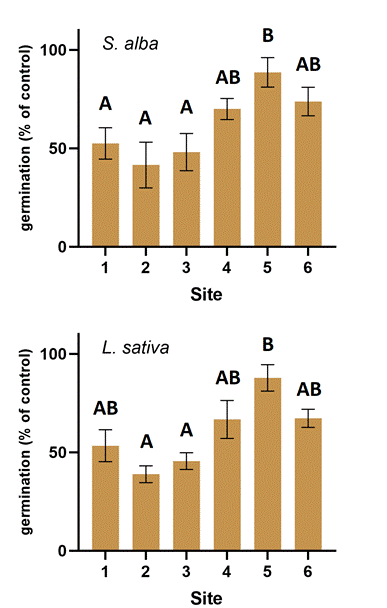
The soil sample from site 2 was the most toxic to the root elongation of the test plants, reducing this parameter by about 40 % (Figure 4.). Soils from sites 4 and 6, on the other hand, did not or only slightly inhibit root elongation. The other samples caused between 10 % and 30 % inhibition .
Overall, there was no clear difference between the sensitivities of the two plant tests for either germination or root elongation.
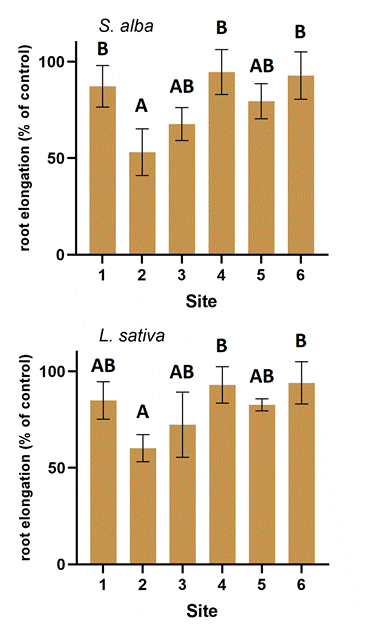
Interestingly, the results of invertebrate tests were inconsistent with the bacterial and plant tests. In the F. candida test, the sample from site 3 proved to be the most toxic in terms of animal survival, killing more than 70% of the test animals compared to the control (Figure 5.). Soil from sites 4 and 5 killed nearly 50 %, while soil from site 6 nearly 40 % of F. candida individuals. The soil sample from sites 1 and 2 was significantly less toxic in this regard, killing less than 20 % of the test animals.
In contrast to the F. candida test, in the E. fetida test, soil samples did not have a large adverse effect on the survival of individuals. They decreased this parameter by less than 10 % compared to the control. Among the samples from different sites, there were no significant differences.
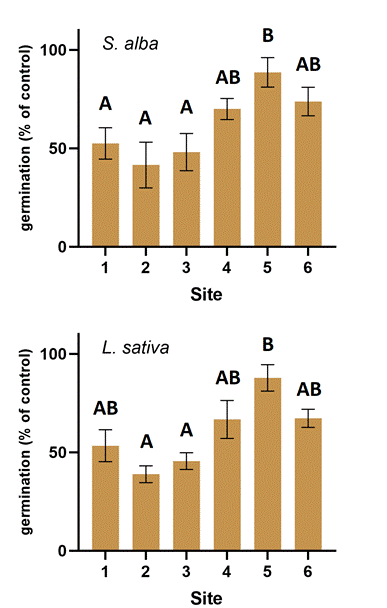
Reproduction was found to be a more sensitive endpoint than survival in both invertebrate tests (Figure 6.). Soil from sites 3, 4, and 5 reduced F. candida reproduction rate by about 80 %, while soil from site 6 also reduced by nearly 60 %. Samples from sites 1 and 2 did not prove to be very toxic in this respect either.
The soil sample from site 5 was also highly toxic to the reproduction rate of E. fetida, decreasing it by more than 70 %. This is significantly more than the result obtained for the sample from sites 1 and 4. The other five samples caused between 15 % and 40 % inhibition in reproduction.
Comparing the two invertebrate tests, it can be concluded that the F. candida test was more sensitive.
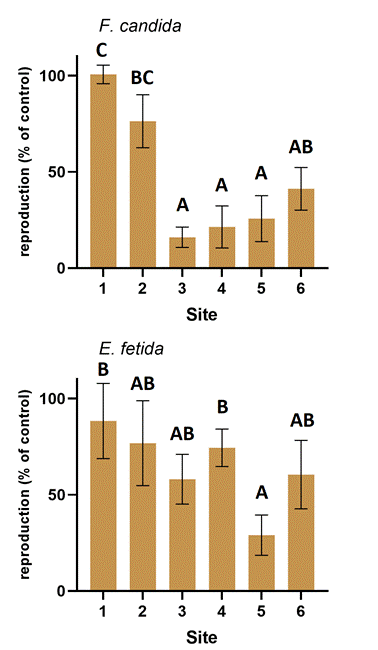
Results of the ecotoxicological characterization indicated poor soil quality since it showed that all tested soil samples had some degree of toxic effects on some test species. It can be concluded that the soil from Site 3 was relatively toxic in almost all ecotoxicological tests. Soils from Sites 1 and 2 were more toxic to bacteria and plants, while soils from sites 4, 5, and 6 were more toxic to the invertebrates. These differences demonstrate that it is not enough to include only one or two species in the studies when assessing soil quality and toxicity. The more species we use, the more accurate our results are likely to be [12][15]. Looking at the endpoints examined, the more sensitive were: DHA of P. fluorescens , germination rates of the test plants, and reproduction rates of test invertebrates.
3.3. Correlation between physico-chemical and ecotoxicological data
Based on Spearmen’s correlation analysis, there was no significant relationship between the examined physico-chemical characteristics and the results of bacterial tests (Table 3.). The only exception was the TS contents of the soil, which was negatively correlated with the IC 50 values obtained from the A. agilis test.
Hty content of the soil samples was positively correlated with the germination rate of S. alba and the root elongation of both plants. This was expected, as it is well known that humus promotes plant growth. In contrast, the Pb concentration of soils was negatively correlated with the germination rate and root elongation of the test plants. Pb is a non-essential element for plants and, according to previous studies, causes significant damage to them in excessive concentrations [21], [22], [23]. This could also have happened in our study, as the Pb concentration of our soil samples was relatively high.
Table 3. Correlation between physico-chemical data and the results of bacterial and plant tests.
Bacterial tests | Plant tests | |||||
IC50 value | germination rate | root elongation | ||||
A. agilis | P. fluorescens | S. alba | L. sativa | S. alba | L. sativa | |
pH | 0.04 | -0.04 | 0.10 | 0.21 | -0.10 | 0.01 |
TS* | -0.49 | -0.20 | -0.26 | 0.31 | 0.08 | 0.02 |
Hty+ | 0.23 | 0.02 | 0.48 | 0.35 | 0.53 | 0.55 |
CaCO3 | 0.06 | 0.15 | -0.03 | -0.14 | 0.13 | 0.20 |
Cd | 0.34 | 0.22 | 0.25 | 0.15 | -0.23 | -0.09 |
Co | 0.29 | 0.10 | 0.32 | 0.35 | -0.02 | 0.03 |
Cr | 0.27 | 0.09 | 0.33 | 0.37 | -0.02 | 0.05 |
Cu | -0.04 | 0.09 | 0.30 | 0.06 | 0.42 | -0.01 |
Ni | 0.35 | 0.03 | 0.41 | 0.43 | 0.43 | 0.36 |
Pb | -0.31 | -0.39 | -0.65 | -0.66 | -0.48 | -0.51 |
Zn | -0.31 | -0.29 | -0.42 | -0.38 | 0.19 | 0.22 |
Data show Spearman’s correlation coefficients. Notes : *Total salt content, +Humus content. Bold numbers indicate a significant correlation (Spearman’s correlation, p < 0.05).
Soil heavy metal concentrations strongly influenced the survival rate and reproduction of F. candida.
Table 4. Correlation between physico-chemical data and the results of invertebrate tests.
Invertebrate tests | ||||
survival rate | reproduction rate | |||
F. candida | E. fetida | F. candida | E. fetida | |
pH | -0.11 | -0.04 | 0.04 | 0.11 |
TS* | -0.26 | 0.07 | -0.21 | -0.08 |
Hty+ | -0.14 | 0.09 | -0.23 | 0.41 |
CaCO3 | -0.22 | -0.06 | -0.30 | 0.11 |
Cd | -0.58 | -0.39 | -0.68 | 0.34 |
Co | -0.71 | -0.10 | -0.71 | 0.36 |
Cr | -0.55 | -0.21 | -0.61 | 0.44 |
Cu | 0.34 | 0.20 | 0.37 | -0.48 |
Ni |
-0.51 | -0.02 | -0.58 | 0.17 |
Pb | 0.45 | 0.38 | 0.53 | -0.19 |
Zn | 0.21 | 0.17 | 0.27 | -0.48 |
Data show Spearman’s correlation coefficients. Notes : *Total salt content, +Humus content. Bold numbers indicate a significant correlation (Spearman’s correlation, p < 0.05).
Concentrations of Cd, Cr, Co, and Ni were significantly correlated with these two parameters, and the correlation coefficients were also relatively high (between -0.51 and -0.71). This suggests that high soil concentrations of these metals may be the reason for the high toxicity of our samples to F. candida. The high sensitivity of this invertebrate to various heavy metals has been established in the past [24]. Interestingly, the Pb concentration of soils was positively correlated with the reproduction rate of F. candida .
None of the physico-chemical parameters examined were statistically related to the survival rate of E. fetida. However, the concentration of Cu and Zn in the soil negatively correlated with its reproduction rate. This means that the reproduction of E. fetida was inhibited by completely different heavy metals than that of F. candida.
4. Conclusion
In our research, we examined the quality and toxicity of suburban soils in Budapest using physico-chemical and ecotoxicological methods. It was revealed that some heavy metals (e.g. Cr, Cr, and Pb) presented in relatively high concentrations in soil samples, which may be the main reason for the observed toxicity of the samples. In fact, all soil samples examined were found to be toxic to at least one test species under laboratory conditions, which means that these soils could be also harmful to terrestrial communities on sites. This draws attention to the necessity of examining suburban soils.
References
[1] S. Norra and D. Stuben, “Urban soils,”Journal of Soils and Sediments, vol. 3, no. 4, pp. 230-233, 2003. View Article
[2] D. Mónok, L. Kardos, S. A. Pabar, Z. Kotroczó, E. Tóth and G. Végvári (2020). “Comparison of soil properties in urban and non‐urban grasslands in Budapest area,” Soil Use and Management, 2020. View Article
[3] L. B. Byrne “Habitat structure: a fundamental concept and framework for urban soil ecology,” Urban Ecosystems, vol. 10, no. 3, pp. 255-274, 2007 View Article
[4] A. Lehmann and K. Stahr, “Nature and significance of anthropogenic urban soils,” Journal of Soils and Sediments, vol. 7, no. 4, pp. 247-260, 2007. View Article
[5] B. Wei and L. Yang, “A review of heavy metal contaminations in urban soils, urban road dusts and agricultural soils from China,” Microchemical journal, vol. 94, no. 2, pp. 99-107, 2010. View Article
[6] Z. Yang, W. Lu, Y. Long, X. Bao and Q. Yang, “Assessment of heavy metals contamination in urban topsoil from Changchun City, China,” Journal of Geochemical Exploration, vol. 108, no. 1, pp. 27-38, 2011. View Article
[7] Y. N. Vodyanitskii, “Contamination of soils with heavy metals and metalloids and its ecological hazard (analytic review),” Eurasian Soil Science, vol. 46, pp. 793-801, 2013. View Article
[8] L. Madrid, E. Dı́az-Barrientos and F. Madrid, “Distribution of heavy metal contents of urban soils in parks of Seville,” Chemosphere, vol. 49, no. 10, pp. 1301-1308, 2002. View Article
[9] W. Burghardt, J. L. Morel and G. L. Zhang, “Development of the soil research about urban, industrial, traffic, mining and military areas (SUITMA),” Soil science and plant nutrition, vol. 61, no. 1, pp. 3-21, 2015. View Article
[10] J. R. Wolch, J. Byrne and J. P. Newell, “Urban green space, public health, and environmental justice: The challenge of making cities ‘just green enough’,” Landscape and urban planning, vol. 125, pp. 234-244, 2014. View Article
[11] C. Haaland and C. K. van den Bosch, “Challenges and strategies for urban green-space planning in cities undergoing densification: A review,” Urban forestry & urban greening, vol. 14, no. 1, pp. 760-771, 2015. View Article
[12] L. Leitgib, J. Kálmán, J. and K. Gruiz, “Comparison of bioassays by testing whole soil and their water extract from contaminated sites,” Chemosphere, vol. 66, no. 3, pp. 428-434, 2007. View Article
[13] P. Alvarenga, P. Palma, A. De Varennes and A. C. Cunha-Queda “A contribution towards the risk assessment of soils from the São Domingos Mine (Portugal): chemical, microbial and ecotoxicological indicators,” Environmental Pollution, vol. 161, pp. 50-56, 2012 View Article
[14] K. Gruiz, V. Feigl, C. Hajdu and M. Tolner, “Environmental toxicity testing of contaminated soil based on microcalorimetry,”Environmental toxicology, vol. 25, no. 5, pp. 479-486, 2010. View Article
[15] V. A. Terekhova, “Soil bioassay: problems and approaches,” Eurasian Soil Science, vol. 44, no. 2, pp. 173-179, 2011. View Article
[16] C. A. van Gestel, J. J. van der Waarde, J. G. Derksen, E. E. van der Hoek, M. F. Veul, S. Bouwens, and G. N. Stokman, “The use of acute and chronic bioassays to determine the ecological risk and bioremediation efficiency of oil‐polluted soils,”Environmental Toxicology and Chemistry: An International Journal, vol. 20, no. 7, pp. 1438-1449, 2001. View Article
[17] E. J. Cardoso and P. R. L. Alves, “Soil Ecotoxicology” in Ecotoxicology, G. Begum, Ed. Rijeka: InTech Europe, 2002, pp. 27-50.
[18] OECD, “Test No. 208: Terrestrial Plant Test: Seedling Emergence and Seedling Growth Test,” in:OECD Guidelines for the Testing of Chemicals, Ed. Paris: OECD Publishing, 2006.
[19] OECD, “Test No. 232: Collembolan Reproduction Test in Soil,” in: OECD Guidelines for the Testing of Chemicals, Ed. Paris: OECD Publishing, 2009.
[20] OECD, “Test No. 222: Earthworm Reproduction Test (Eisenia fetida/Eisenia andrei),” in: OECD Guidelines for the Testing of Chemicals, Ed. Paris: OECD Publishing, 2016. View Article
[21] P. C. Nagajyoti, K. D. Lee and T. V. M. Sreekanth, “Heavy metals, occurrence and toxicity for plants: a review,” Environmental chemistry letters, vol. 8, no. 3, pp. 199-216, 2010. View Article
[22] P. Sharma and R. S. Dubey “Lead toxicity in plants,” Brazilian journal of plant physiology, vol. 17, no. 1, pp. 35-52, 2005. View Article
[23] F. Hadi and T. Aziz “A mini review on lead (Pb) toxicity in plants,” Journal of Biology and Life Science, vol. 6, no. 2, pp. 91-101, 2015. View Article
[24] M. Liu, J. Xu, P. H. Krogh, J. Song, L. Wu, Y. Luo, and X. Ke“Assessment of toxicity of heavy metal-contaminated soils toward Collembola in the paddy fields supported by laboratory tests,” Environmental Science and Pollution Research, vol. 25, no. 17, pp. 16969-16978, 2018. View Article