Volume 5 - Year 2017 - Pages 1-14
DOI: 10.11159/ijepr.2017.001
Metal Accumulation and Metallothionein Response in Fucus Spiralis
J. F. P. Oaten1, M. C. Gibson1, M. D. Hudson1, A. C. Jensen2, I. D. Williams1
1 Centre for Environmental Science, Faculty of Engineering and the Environment, University of Southampton
University Road, Highfield, Southampton, Hampshire, SO17 1BJ, United Kingdom
Jfpo1g13@soton.ac.uk; Mcg1g13@soton.ac.uk; Mdh@soton.ac.uk; Idw@soton.ac.uk
2 Ocean and Earth Science, University of Southampton, Waterfront Campus, National Oceanography Centre
European Way, Southampton, Hampshire, SO14 3ZH, United Kingdom
Acj@noc.soton.ac.uk
Abstract - Seaweeds are established sentinels for metal contamination and are utilised for biomonitoring. Metallothionein (MT) is a protein that is induced by metal exposure, and has been widely used as a biomarker for metal pollution. MT has not been reported in spiral wrack (Fucus spiralis), but has been identified in bladder wrack (Fucus vesiculosus), where it has been suggested to be a protective mechanism against metal exposure. This study aimed to evaluate the potential use of MT in F. spiralis as a biomarker for metal pollution for the first time. Samples were collected from Poole Harbour, UK, over a year-long period in 2015. MT and metal concentrations were quantified during winter, spring, summer, and autumn. Linear regression analysis showed few relationships between MT and metal concentrations throughout most seasons. However, during summer, significant positive relationships existed between MT concentrations and iron (R2= 0.631), nickel (R2 = 0.486), tin (R2 = 0.579), and lead (R2 = 0.415). Furthermore, MT concentrations were significantly higher in summer than in other seasons. It is possible that for most of the year, metal concentrations in Poole Harbour are not high enough to elicit a MT response in F. spiralis, as it is a metal tolerant species. This suggests that the use of MT as a sensitive biomarker for metal pollution in F. spiralis, at low levels of metal pollution, is limited. However, increased toxicity of metals, and vulnerability of seaweeds to metals, may become pertinent in summer months as metals inhibit photosynthetic processes and growth. This may have caused MT to relate to metal concentrations more closely during summer, as it responds to the increased effects of metals. Therefore, the potential for using MT in F. spiralis as a biomarker for metal pollution may be restricted to summer months in temperate regions, at least at low metal concentrations. Further research is required to fully evaluate the use of MT in Fucus spp., addressing uncertainties of seasonal variation, and MT response in severely polluted environments.
Keywords: Metallothionein, metal toxicity, biomonitoring, brown seaweed, Fucus spiralis
© Copyright 2017 Authors - This is an Open Access article published under the Creative Commons Attribution License terms. Unrestricted use, distribution, and reproduction in any medium are permitted, provided the original work is properly cited.
Date Received: 2016-09-19
Date Accepted: 2017-03-14
Date Published: 2017-12-01
1. Introduction
Biomonitoring, whereby organisms are used to measure environmental pollution, has advantages over direct measurements of chemical contaminants in the environment, such as seawater or sediment concentrations. It provides an exclusive measure of the bioavailable pollutants, which have the greatest potential to impact organisms, ecosystems, and human health, and discounts pollutants not taken up by organisms [1]. Furthermore, it allows the measurement of pollutants accumulated over an organism’s lifetime, which reduces fluctuations in pollutant concentrations [2]. Previously, the environmental quality of water bodies was assessed using chemical monitoring alone, missing information on ecological impacts and overall water quality [3]. Under the Water Framework Directive (WFD 2000/60/EC), water bodies should be assessed through both chemical and ecological monitoring [4].
Seaweeds are advocated as bioindicators in temperate coastal waters due mainly to their high abundances and immobility [5]. They often dominate metal contaminated habitats [6] as they are resistant to metal pollution [7]. They have an ability to accumulate dissolved metals from seawater so their intracellular concentrations reflect time-integrated pollution loads in the marine environment [8]. As a consequence, seaweeds are established sentinels for metal contamination and are exploited for biomonitoring [9].
Metallothionein (MT) is a protein of low molecular weight, high heat stability, and high cysteine content [10]. The latter attribute lends itself to be used as a biomarker of metal pollution, as it has a high affinity to bond to metals due to sulphur-containing thiol groups [11]. It is regarded to play a vital role in the detoxification of metals within organisms [11, 12]. This relays a biological response indicating the severity of metal pollution to the organism. Many organisms have been employed as a MT biomarker species, primarily bivalve species [13-17]. However, MT is also noted to have multiple roles such as maintaining homeostasis by regulating essential metals, and as a defence against reactive oxygen species [18, 19]. Factors that contribute to natural variation of MT include tissue weight [20], reproductive stage [21], temperature [22], and salinity [23]. This limits the use of MT as a tool in biomonitoring, as concentrations may alter independently of metal exposure, particularly in bivalve species.
Literature on the MT response in marine algae to metal exposure is limited, compared to other organisms. MT in spiral wrack (Fucus spiralis) has never been reported. The MT gene has been identified in bladder wrack (Fucus vesiculosus) by Morris et al. [24], which suggested that a protective mechanism against metal exposure exists for this species. Further studies suggest induction in this species following Cu exposure [9], as well as an ability to bind to As, Cd, and Zn [25, 26]. This shows potential for MT to be developed as a biomarker in brown seaweeds.
F. spiralis would offer a cosmopolitan bioindicator species for dissolved metal pollution, if MT is shown to be a reliable biomarker in this species. It is geographically widely available and easy to sample, suggesting it is a promising candidate. It may also be less susceptible to natural variation compared to traditional MT biomarker species. However, despite the potential for F. spiralis to be used as a MT biomarker species, its use has not been developed, and no study of its MT response to metals has been conducted in the field. Therefore, this study aimed to investigate the potential for MT in F. spiralis to be used as a biomarker for metal pollution.
2. Methodology
2.1. Sample collection
Seaweed samples were collected from four sites in Poole Harbour, UK: Holes Bay (north), Holes Bay (south), Poole Quay, and Sandbanks (Figure 1). Samples were carried out in January, April, August, and October, which are referred to as winter, spring, summer, and autumn. Samples were kept in storage at -20 °C before analysis, as advised by Oaten, et al. [27].
2.2. MT analysis
MT concentrations were measured using the UV-spectrophotometric method devised by Viarengo, et al. [28], with modifications by Aly, et al. [29]. Before analysis, approximately 3 cm of the frond tips of seaweed were dissected. This was then homogenised using a ceramic blade and a pestle and mortar (to avoid metal contamination before metal analysis). Three replicates of each sample were measured. Concentrations are reported in µg/g (wet weight).
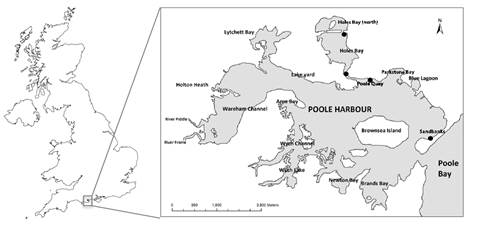
2.3. Metal analysis
Before analysis, previously homogenised samples (as per MT analysis) were freeze-dried for 72 hours. Accurately weighed samples of approximately 10 mg of dried, ground sample were placed in 7 ml Teflon sealable pots. Blank samples consisting of empty Teflon pots were also prepared. Samples were digested in Aqua Regia on a hot plate. Additions of trace metal grade hydrogen peroxide (H2O2) were made to oxidize organic matter. Samples were dried, resuspended, and completed with 3% trace metal grade, redistilled, nitric acid (HNO3), containing 5 ppb In/Re and 20 ppb Be as internal standards to correct for matrix effects and instrument drift. Analysis by inductively coupled plasma mass spectrometry (ICP–MS) was carried out. A mussel reference material (European Reference Materials – CE278k) was measured as a bivalve comparator and concentrations were adjusted according to the recovery rate. Concentrations are reported as µg/g (dry weight).
2.4. Statistical analysis
All statistical analysis was completed using IBM SPSS Statistic v21. Tests for normality (Shapiro-Wilk) and homogeneity of variance (Levene’s test) were completed and data was tested parametrically (one-way ANOVA) or non-parametrically (Kruskall-Wallis test), accordingly. Linear regression was used to determine the effects of metal exposure on MT concentrations in F. spiralis. Statistical significance was established at P = 0.05.
3. Results
MT concentrations in F. spiralis varied greatly throughout the sampling year (Figure 2a, Table 1). In winter, MT concentrations were significantly higher in Holes Bay (north), compared to Holes Bay (south), Poole Quay, and Sandbanks (post-hoc Scheffe, P = 0.015, P < 0.001, P < 0.001, respectively). Holes Bay (south) was also significantly higher than Poole Quay and Sandbanks (post-hoc Scheffe, P = 0.004, P = 0.048, respectively). Concentrations of MT in F. spiralis from Sandbanks increased in spring, and became highest in summer and autumn. In spring, MT concentrations were higher in Holes Bay (north) compared to Poole Quay (post-hoc Tukey, P = 0.04). In summer, the concentration of MT in F. spiralis from Sandbanks was significantly higher than Poole Quay (post-hoc Scheffe, P = 0.026). During autumn, significant differences in MT concentrations in F. spiralis did not exist between sites (F = 2.835, P = 0.106). Across seasons, mean MT concentrations in winter were significantly lower than concentrations in spring (post-hoc Scheffe, P = 0.047), summer (post-hoc Scheffe, P = 0.016), and autumn (post-hoc Scheffe, P = 0.015) (Table 1).
Metal concentrations in F. spiralis also varied greatly throughout the sampling year, and were inconsistent in each season (Figure 2b – j, Table 1). For Fe, Ag and Cd, highest concentrations were generally found at Holes Bay (north) throughout the year. For Sn and Ni concentrations were highest at Sandbanks in winter and summer, and highest at Holes Bay (north) in spring and autumn. For Zn and As, highest concentrations were predominantly found at Holes Bay (south), and for Cu during winter and spring. Pb concentrations were highest in F. spiralis at Holes Bay (north), Poole Quay, and Sandbanks in winter, spring, and summer, respectively. Furthermore, concentrations of Cu, Zn, As, Ag, Sn and Cd generally decrease from winter to autumn (Table 1). Across seasons, mean Zn, As, Ag, and Cd concentrations were significantly different (Table 1). Winter As concentrations were significantly higher than concentrations in spring (post-hoc Scheffe, P = 0.006), summer (post-hoc Scheffe, P < 0.001) and autumn (post-hoc Scheffe, P < 0.001). In winter, Zn and Ag concentrations were significantly higher than in summer (post-hoc Scheffe, P < 0.001, P = 0.003, respectively) and autumn (post-hoc Scheffe, P = 0.002, P = 0.004, respectively). Cd concentrations were significantly higher in winter than summer (post-hoc Scheffe, P = 0.002).
Linear regression analysis was used to assess the effect of metal exposure on MT concentration. During winter, only Fe tissue concentration showed a significant positive relationship with MT concentration (R2 = 0.792, P < 0.001) (Figure 3a). In summer, significant positive relationships were evident, and existed between MT concentrations and Fe (R2= 0.631, P = 0.002) (Figure 3a), Ni (R2 = 0.486, P = 0.012) (Figure 3b), Sn (R2 = 0.579, P = 0.004) (Figure 3c), and Pb (R2 = 0.415, P = 0.024) (Figure 3d). No significant positive relationships existed between MT and any other metals, during any season, and are therefore not shown graphically.
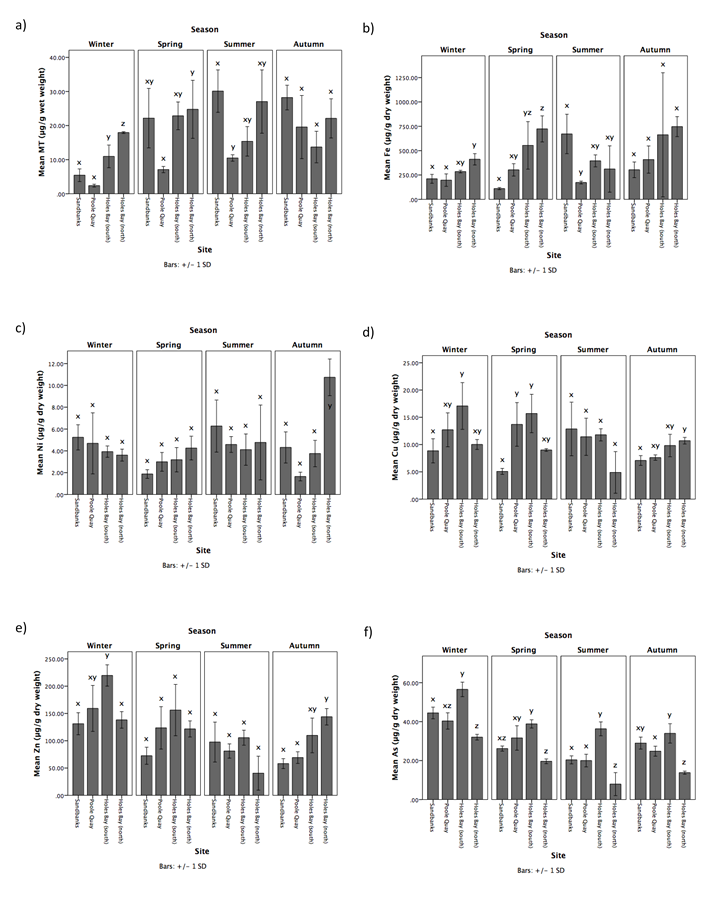
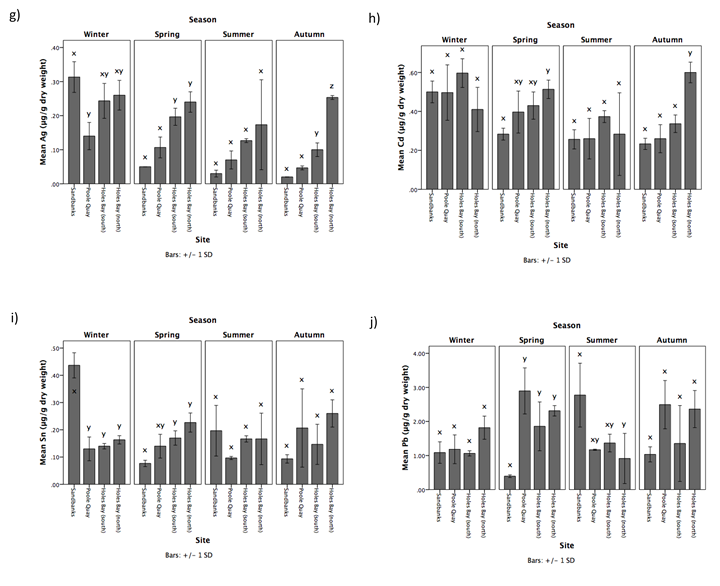
Table 1. Mean seasonal concentrations of MT (μg/g wet weight) and metals (μg/g dry weight) in F. spiralis sampled from Holes Bay (north), Holes Bay (south), Poole Quay, and Sandbanks in Poole Harbour in 2015. Different letters (x, y, z) indicate significant differences between seasons (P = 0.05).
Winter | Spring | Summer | Autumn | ||
MT (μg/g) | Mean | 9.18 x | 19.22 y | 20.75 y | 20.90 y |
SD | 6.40 | 9.20 | 9.87 | 7.58 | |
Fe (μg/g) | Mean | 276.08 x | 422.60 x | 387.71 x | 530.29 x |
SD | 98.16 | 273.29 | 233.55 | 340.85 | |
Ni (μg/g) | Mean | 4.37 x | 3.08 x | 4.94 x | 5.11 x |
SD | 1.49 | 1.18 | 2.09 | 1.07 | |
Cu (μg/g) | Mean | 12.17 x | 10.87 x | 10.24 x | 8.81 x |
SD | 4.13 | 4.88 | 4.48 | 1.87 | |
Zn (μg/g) | Mean | 162.07 x | 118.52 xy | 81.28 y | 95.29 y |
SD | 42.75 | 41.66 | 34.20 | 39.05 | |
As (μg/g) | Mean | 43.34 x | 29.07 y | 21.15 y | 25.40 y |
SD | 9.64 | 7.93 | 11.06 | 8.23 | |
Ag (μg/g) | Mean | 0.24 x | 0.15 xy | 0.10 y | 0.11 y |
SD | 0.08 | 0.08 | 0.08 | 0.09 | |
Cd (μg/g) | Mean | 0.50 x | 0.41 xy | 0.29 y | 0.36 xy |
SD | 0.11 | 0.10 | 0.11 | 0.16 | |
Sn (μg/g) | Mean | 0.22 x | 0.15 x | 0.16 x | 0.18 x |
SD | 0.14 | 0.06 | 0.07 | 0.10 | |
Pb (μg/g) | Mean | 1.29 x | 1.87 x | 1.56 x | 1.81 x |
SD | 0.42 | 1.06 | 0.92 | 0.90 |
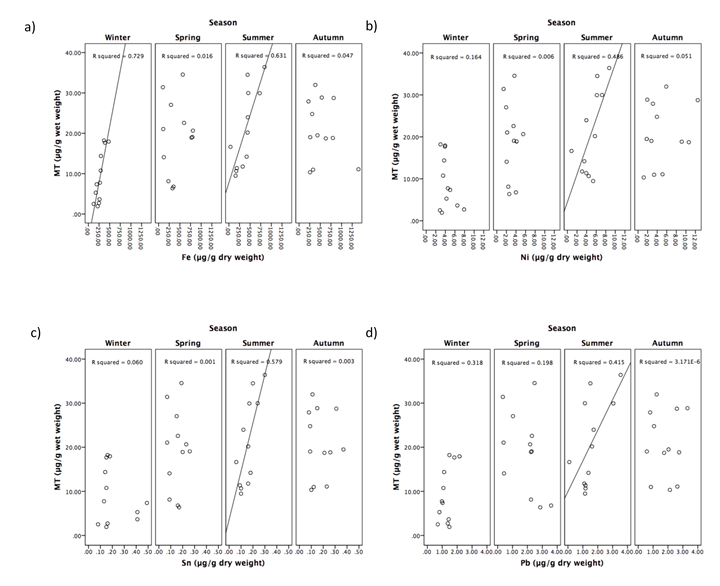
4. Discussion
4.1. Metal contamination and seasonal variation
Concentrations of metals in F. spiralis from Poole Harbour indicate that the most polluted area is Holes Bay (Figure 2b - j). This is in agreement with previous literature on metal pollution in Poole Harbour by Langston et al. [30], Aly et al. [31], and Oaten et al. [32], which reported generally higher metal concentrations within Holes Bay in sediment and seawater, as well as biota. F. spiralis metal concentrations at Sandbanks were also relatively high. This may be due to the sewage pumping station near to the site, which periodically discharges storm water. There are also yacht clubs in the vicinity, which may contribute to the metal burden in the area due to sources of metals such as anti-fouling paints on watercraft: higher Sn concentrations in winter could be related to boat maintenance in winter and the removal of old tributyl tin antifoulant.
Seasonal variation for some metal concentrations in F. spiralis is apparent. Cu, Zn, As, Ag, Cd, and Sn concentrations tend to reduce from winter to autumn (Table 1). This could be explained by plant growth. Concentrations within seaweeds continue to mount through dormant periods during winter, and dilute as plants grow and reproduce in summer months [33, 34]. Metal bioavailability may also influence seasonal variability of bioaccumulated metal concentrations. For example, dissolved Cd concentration tends to reduce during summer months due to uptake by phytoplankton [35]. Furthermore, higher salinity, which is observed during warmer months due to reduced rainfall and increased evaporation, also causes increased rates of Cd, Zn and Cu binding to chloride ions, thereby reducing dissolved metal bioavailability [36, 37]. Therefore, because seaweeds are predominantly exposed to dissolved metals concentrations within seawater, as they inhabit the water column, Cd, Cu and Zn exposure to seaweeds is reduced during summer. Seasonal variation in brown seaweed metal concentrations has been shown to be particularly evident for Cd and Zn, but less difference is observed for Pb and Ni [38, 39]. This may be explained by an ion-exchange process that actively takes up Pb and Ni, meaning the importance of bioavailability on bioaccumulation of these metals is less [40-42]. Furthermore, the process of phytoplankton metal uptake during summer, and salinity effects to dissolved metal bioavailability is rarely reported for Pb or Ni. This may explain the reduced seasonal variability in Pb and Ni F. spiralis concentrations observed in this study (Table 1).
4.2. Metal toxicity and MT induction
Generally, the induction of MT is a physiological response to the insult caused by metal exposure [24, 25]. Therefore, toxic metals are more likely to cause MT induction. The order of toxicity of metals to seaweed species is generally Hg > Cu > Cd > Ag > Pb > Zn [43]. Cu, despite being an essential metal, is the second most toxic metal to seaweeds, the effects of which have been extensively studied due to its use in antifouling paints [43, 44]. It is often cited to inhibit photosynthetic processes and retard growth in seaweed species [45-47]. In addition, inhibition of fertilization and reproduction resulting from Cu exposure has been identified in F. spiralis [48]. Pb has also been shown to impact photosynthetic efficiency and growth of red and brown seaweeds, but is less toxic than Cu [45, 49]. Cd can affect growth, pigment content, and carbon assimilation in seaweeds [50]. Zn has also been shown to slow growth in seaweeds [51].
There is limited knowledge on MT response to metal exposure in seaweeds, though few studies exist on F. vesiculosus. Morris, et al. [24] noted the MT gene in F. vesiculosus to be induced by Cu exposure, and that MT can bind to both Cu and Cd. Further studies confirmed its role as a detoxification mechanism for metals, and reported MT binding abilities to Zn, and As [25, 26]. However, only Owen, et al. [9] confirmed this role in the field in vivo. The study found MT to respond to Cu exposure, and this metal was found to be more important for MT induction, due to a stronger and more significant regression coefficient, compared to Zn and Fe. In this study, Cu, Zn, and As seemed not to elicit a MT response in F. spiralis. Furthermore, during summer these metals were at minimal concentrations in F. spiralis when MT concentrations were at a maximum (Table 1). However, it is important to note that metal body burdens (total mass of metals within the organism) of these metals are likely to be less sensitive to change. This is due to metal uptake increases with increased metabolic rates in response to temperature and light increases, and subsequent growth rate increases, in spring and summer [42, 52]. Fe, Ni, Sn and Pb showed a significant positive relationship with MT in F. spiralis, during summer. Previous studies have not reported MT induction in Fucus spp. following exposure from these metals, with the exception of Fe [9]. However, these metals are known to induce MT in other species [53, 54]. Otherwise, it is possible these metals are contributing to a combination effect with other more toxic metals, such as Cu, and are cumulatively above a threshold for MT induction [55]. Another possibility is that these metals are correlated with more toxic metals, not recorded here, that are eliciting a MT response in F. spiralis.
4.3. Influences of MT response and variability
MT concentrations in F. spiralis were generally low for most of the year and were not related to tissue metal concentrations. This may be due to relatively low levels of metal exposure in Poole Harbour [31]. Seaweed species are very tolerant of metal exposure [7]. As such, the concentrations in Poole Harbour may not be enough to elicit a MT response. A study by Owen, et al. [9] reported F. vesiculosus to begin exhibiting the gene for MT when exposed to a concentration of Cu of 30 µg/l. However, Cu concentrations in seawater in Poole Harbour do not exceed 3 µg/l [31]. For comparison, Zn, Fe, and Pb concentrations in F. vesiculosus from the Fal Estuary, Cornwall, were as much as an order of magnitude higher, with Cu two orders of magnitudes higher, compared to this study [56]. Owen, et al. [9] did not report tissue concentrations as high in F. vesiculosus from the Fal Estuary; perhaps indicating a recovery of contamination levels, but the most polluted site studied was still approximately ten, five, and ten times higher respectively for Cu, Zn and Fe concentrations than in F. spiralis in this study.
Aside from low seawater concentrations, low accumulation of metals in seaweeds from Poole Harbour may be the product of low metal concentrations in the tips of fronds, with greater concentrations in the thallus [56]. It has been suggested to dissect the frond at a pre-determined distance from the distal end (10 cm for F. vesiculosus) to allow time for new growth to equilibrate with the environment [57, 58]. In this study, tips of seaweeds were analysed in order to select the tissue that reflected the most recent metal concentrations in the surrounding water [46]. However, it may be more suitable to analyse metal exposure and MT response in mature tissue in the thallus, due to potentially higher metal, and likely MT, concentrations. This may also explain the large degree of variation in metal and MT concentrations, evidenced by large standard deviations.
Biological processes may provide insight as to why metals only seem to elicit a MT response in this species during summer. It is known that prolonged exposure to metals can cause damage to growth rates and photosynthetic efficiency in seaweeds [45]. This is likely due to the redirection of energy for defensive pathways to protect against metals, allowing less energy for growth [45]. The oxidation of photosynthetic pigments by Cu, for example, may also cause a reduction in photosynthetic capability, which also causes production of reactive oxygen species leading to oxidative stress [59, 60]. Furthermore, the substitution of Mg by other metals within chlorophyll can inhibit photosystem II, the reaction centre for capturing photons for photosynthesis, leading to chloroplast dysfunction and photosynthetic processes to cease [47]. Pb, Zn, and Cu can also affect Photosystem II in plants, by inhibiting electron transport and altering the structure of the thylakoid membrane within chloroplasts, whilst Cd and Ni can interact with other metabolic processes [61]. Both photosynthesis and growth rates are at a maximum during summer. Therefore, it is logical to suggest the affect of metals on these processes is exacerbated during summer, causing metal toxicity to seaweeds to increase. MT has been shown to protect against oxidative stress through oxyradical scavenging [18]. MT may therefore follow metal concentrations more closely in summer, due to increased toxicity and oxyradical production caused by metal affects on photosynthesis and growth rates. This could explain the positive relationship between seaweed metal concentrations and MT during summer in this study (Figure 3).
Nutrient concentrations may also impact metal toxicity. Addition of nitrogen and phosphorus has been shown to reduce metal toxicity to Cd, allowing growth rates of red macroalga (Gracilaria tenuistipitata) to increase [59, 62]. This may be caused by nutrient complexation with metals, competition of uptake sites with metals, and improved nutrient status of plant organisms [62]. Nutrient concentrations within Poole Harbour towards the end of summer are likely to be minimal [63]. This is due to reduced rainfall and consequent reduction in land based inputs of nitrogen and phosphorus [64]. Increased uptake of nutrients by primary production throughout spring and summer also further reduces nutrient concentrations in the water column [65]. Therefore, low nutrient concentrations in summer may further be increasing the vulnerability of F. spirals to metal toxicity, possibly resulting in a MT response.
5. Conclusion
The use of MT in F. spiralis as a sensitive biomarker of metal pollution at low concentrations, as subjected in Poole Harbour, is shown here to be limited, as MT does not appear to be consistently induced by metal exposure. It is possible that for most of the year, metal concentrations in Poole Harbour are not high enough to elicit a MT response in F. spiralis, as it is a metal tolerant species. However, during summer, concentrations of MT increase, and linear regression analysis reveals significant positive relationships with Ni, Pb, Sn, and Fe. This may be due to increased toxicity of metals, and vulnerability of seaweeds to metals, as they inhibit photosynthetic processes and growth, which becomes pertinent in summer months. This may have caused MT to relate to metal concentrations more closely, as it responds to the increased effects of metals. This shows evidence for MT in F. spiralis to be able to relay the biological impact of metals at low metal concentrations during summer, when important physiological processes are taking place, such as photosynthesis. Therefore, the potential for using MT in F. spiralis as a biomarker for metal pollution may be restricted to summer months in temperate regions, at least at low metal concentrations. Further research is required to fully evaluate MT response in Fucus spp., addressing uncertainties in frond selection, and seasonal variation and effects. MT response in Fucus spp. in severely polluted environments should also be examined in order to establish its relevance as a cosmopolitan bioindicator species.
Acknowledgements
The authors would like to thank the Engineering and Physical Sciences Research Council (EP/L505067/1) and the Southampton Marine and Maritime Institute (SMMI) for jointly financing this study. Thanks are also due to Professor John Humphreys, for assistance with field sampling, and Dr Matthew Cooper for laboratory assistance and advice.
References
[1] W. J. Langston, S. O'Hara, N. D. Pope, M. Davey, E. Shortridge, M. Imamura, H. Harino, A. Kim, and C. H. Vane, "Bioaccumulation surveillance in Milford Haven Waterway," Environmental Monitoring and Assessment, vol. 184, pp. 289-311, 2012. View Article
[2] J. E. Marcovecchio, "The use of Micropogonias furnieri and Mugil liza as bioindicators of heavy metals pollution in La Plata river estuary, Argentina," Science of the Total Environment, vol. 323, pp. 219-26, 2004. View Article
[3] J. A. Hagger, T. S. Galloway, W. J. Langston, and M. B. Jones, "Application of biomarkers to assess the condition of European Marine Sites," Environmental Pollution, vol. 157, pp. 2003-2010, 2009. View Article
[4] J. A. Hagger, M. B. Jones, D. Lowe, D. R. P. Leonard, R. Owen, and T. S. Galloway, "Application of biomarkers for improving risk assessments of chemicals under the Water Framework Directive: A case study," Marine Pollution Bulletin, vol. 56, pp. 1111-1118, 2008. View Article
[5] P. S. Rainbow, "Biomonitoring of heavy metal availability in the marine environment," Marine Pollution Bulletin, vol. 31, pp. 183-192, 1995. View Article
[6] H. D. Nielsen and S. L. Nielsen, "Photosynthetic responses to Cu2+ exposure are independent of light acclimation and uncoupled from growth inhibition in Fucus serratus (phaeophyceae)," Marine Pollution Bulletin, vol. 51, pp. 715-721, 2005. View Article
[7] B. Pawlik-Skowronska, J. Pirszel, and M. T. Brown, "Concentrations of phytochelatins and glutathione found in natural assemblages of seaweeds depend on species and metal concentrations of the habitat," Aquatic Toxicology, vol. 83, pp. 190-199, 2007. View Article
[8] J. A. Vasquez and N. Guerra, "The use of seaweeds as bioindicators of natural and anthropogenic contaminants in northern Chile," Hydrobiologia, vol. 327, pp. 327-333, 1996. View Article
[9] J. R. Owen, C. A. Morris, B. Nicolaus, J. L. Harwood, and P. Kille, "Induction of expression of a 14-3-3 gene in response to copper exposure in the marine alga, Fucus vesiculosus," Ecotoxicology, vol. 21, pp. 124-138, 2012. View Article
[10] J. H. R. Kagi and Y. Kojima, "Chemistry and biochemistry of metallothionein," Biochemistry, vol. 27, pp. 8509-8515, 1987.
[11] J. C. Amiard, C. Amiard-Triquet, S. Barka, J. Pellerin, and P. S. Rainbow, "Metallothioneins in aquatic invertebrates: Their role in metal detoxification and their use as biomarkers," Aquatic Toxicology, vol. 76, pp. 160-202, 2006. View Article
[12] C. D. Klaassen, J. Liu, and S. Choudhuri, "Metallothionein: An intracellular protein to protect against cadmium toxicity," Annual Review of Pharmacology and Toxicology, vol. 39, pp. 267-294, 1999. View Article
[13] M. J. Bebianno and W. J. Langston, "Metallothionein induction in Mytilus edulis exposed to cadmium," Marine Biology, vol. 108, pp. 91-96, 1991. View Article
[14] N. Geng, C. Wang, P. F. Wang, N. Qi, and L. X. Ren, "Cadmium accumulation and metallothionein response in the freshwater bivalve Corbicula fluminea under hydrodynamic conditions," Biological Trace Element Research, vol. 165, pp. 222-232, 2015. View Article
[15] S. N. Frank, C. Singer, and B. Sures, "Metallothionein (MT) response after chronic palladium exposure in the zebra mussel, Dreissena polymorpha," Environmental Research, vol. 108, pp. 309-314, 2008. View Article
[16] A. Serafim and M. J. Bebianno, "Kinetic model of cadmium accumulation and elimination and metallothionein response in Ruditapes decussatus," Environmental Toxicology and Chemistry, vol. 26, pp. 960-969, 2007. View Article
[17] A. Geffard, J. C. Amiard, and C. Amiard-Triquet, "Use of metallothionein in gills from oysters (Crassostrea gigas) as a biomarker: seasonal and intersite fluctuations," Biomarkers, vol. 7, pp. 123-137, 2002. View Article
[18] A. Viarengo, B. Burlando, N. Ceratto, and I. Panfoli, "Antioxidant role of metallothioneins: A comparative overview," Cellular and Molecular Biology, vol. 46, pp. 407-417, 2000. View Article
[19] T. T. Le, S. Zimmermann, and B. Sures, "How does the metallothionein induction in bivalves meet the criteria for biomarkers of metal exposure?," Environmental Pollution, vol. 212, pp. 257-68, 2016. View Article
[20] B. Raspor, Z. Dragun, M. Erk, D. Ivankovic, and J. Pavicic, "Is the digestive gland of Mytilus galloprovincialis a tissue of choice for estimating cadmium exposure by means of metallothioneins?," Science of the Total Environment, vol. 333, pp. 99-108, 2004. View Article
[21] V. Moschino, E. Delaney, and L. Da Ros, "Assessing the significance of Ruditapes philippinarum as a sentinel for sediment pollution: Bioaccumulation and biomarker responses," Environmental Pollution, vol. 171, pp. 52-60, 2012. View Article
[22] W. Smaoui-Damak, B. Berthet, and A. Hamza-Chaffai, "In situ potential use of metallothionein as a biomarker of cadmium contamination in Ruditapes decussatus," Ecotoxicology and Environmental Safety, vol. 72, pp. 1489-1498, 2009. View Article
[23] S. Legras, C. Mouneyrac, J. C. Amiard, C. Amiard-Triquet, and P. S. Rainbow, "Changes in metallothionein concentrations in response to variation in natural factors (salinity, sex, weight) and metal contamination in crabs from a metal-rich estuary," Journal of Experimental Marine Biology and Ecology, vol. 246, pp. 259-279, 2000. View Article
[24] C. A. Morris, B. Nicolaus, V. Sampson, J. L. Harwood, and P. Kille, "Identification and characterization of a recombinant metallothionein protein from a marine alga, Fucus vesiculosus," Biochemical Journal, vol. 338, pp. 553-560, 1999. View Article
[25] M. E. Merrifield, J. Chaseley, P. Kille, and M. J. Stillman, "Determination of the Cd/S cluster stoichiometry in Fucus vesiculosus metallothionein," Chemical Research in Toxicology, vol. 19, pp. 365-375, 2006. View Article
[26] T. T. Ngu, J. A. Lee, M. K. Rushton, and M. J. Stillman, "Arsenic metalation of seaweed Fucus vesiculosus metallothionein: the importance of the interdomain linker in metallothionein," Biochemistry, vol. 48, pp. 8806-8816, 2009. View Article
[27] J. F. Oaten, M. D. Hudson, A. C. Jensen, and I. D. Williams, "Effects of organism preparation in metallothionein and metal analysis in marine invertebrates for biomonitoring marine pollution," Science of the Total Environment, vol. 518-519, pp. 238-47, 2015. View Article
[28] A. Viarengo, E. Ponzano, F. Dondero, and R. Fabbri, "A simple spectrophotometric method for metallothionein evaluation in marine organisms: An application to Mediterranean and 12 Antarctic molluscs," Marine Environmental Research, vol. 44, pp. 69-84, 1997. View Article
[29] W. Aly, I. D. Williams, and M. D. Hudson, "Limitations of metallothioneins in common cockles (Cerastoderma edule) and sponges (Haliclona oculata) as biomarkers of metal contamination in a semi-enclosed coastal area," Science of the Total Environment, vol. 473, pp. 391-397, 2014. View Article
[30] W. J. Langston, B. S. Chesman, G. R. Burt, S. J. Hawkins, J. Readman, and P. Worsfold, "Site characterization of the South West European Marine sites - Poole Harbour SPA," Plymouth Marine Science Partnership on behalf of the Environment Agency and English Nature, 2003. View Article
[31] W. Aly, I. D. Williams, and M. D. Hudson, "Metal contamination in water, sediment and biota from a semi-enclosed coastal area," Environmental Monitoring and Assessment, vol. 185, pp. 3879-3895, 2013. View Article
[32] J. F. P. Oaten, M. D. Hudson, A. C. Jensen, and I. D. Williams, "Seasonal effects to metallothionein responses to metal exposure in a naturalised population of Ruditapes philippinarum in a semi-enclosed estuarine environment," Science of the Total Environment, vol. 575, pp. 1279-1290, 2017. View Article
[33] R. Fuge and K. H. James, "Trace metal concentrations in Fucus from the Bristol Channel," Marine Pollution Bulletin, vol. 5, pp. 9-12, 1974. View Article
[34] R. Villares, P. X, and A. Carballeira, "Seasonal variation and background levels of heavy metals in two green seaweeds," Environmental Pollution, vol. 119, pp. 79-90, 2002. View Article
[35] C. Pohl, G. Kattner, and M. Schulzbaldes, "Cadmium, copper, lead and zinc on transects through Arctic and Eastern Atlantic surface and deep waters," Journal of Marine Systems, vol. 4, pp. 17-29, 1993. View Article
[36] G. W. Bryan and W. J. Langston, "Bioavailability, accumulation and effects of heavy metals in sediments with special reference to United Kingdom estuaries - a review," Environmental Pollution, vol. 76, pp. 89-131, 1992. View Article
[37] P. S. Rainbow, "Physiology, physicochemistry and metal uptake - a crustacean perspective," Marine Pollution Bulletin, vol. 31, pp. 55-59, 1995. View Article
[38] F. Riget, P. Johansen, and G. Asmund, "Natural seasonal variation of cadmium, copper, lead and zinc in brown seaweed (Fucus vesiculosus)," Marine Pollution Bulletin, vol. 30, pp. 409-413, 1995. View Article
[39] P. Miramand and D. Bentley, "Heavy metal concentrations in 2 biological indicators (Patella vulgata and Fucus serratus) collected near the French nuclear fuel reprocessing plant of La Hague," Science of the Total Environment, vol. 111, pp. 135-149, 1992. View Article
[40] I. Eide, S. Myklestad, and S. Melsom, "Long-term uptake and release of heavy metals by Ascophyllum nodosum (L.) le jol. (phaeophyceae) in situ," Environmental Pollution Series A, Ecological and Biological, vol. 23, pp. 19-28, 1980. View Article
[41] I. Sanchez-Rodriguez, M. A. Huerta-Diaz, E. Choumiline, O. Holguin-Quinones, and J. A. Zertuche-Gonzalez, "Elemental concentrations in different species of seaweeds from Loreto Bay, Baja California Sur, Mexico: implications for the geochemical control of metals in algal tissue," Environmental Pollution, vol. 114, pp. 145-160, 2001. View Article
[42] V. Besada, J. M. Andrade, F. Schultze, and J. J. Gonzalez, "Heavy metals in edible seaweeds commercialised for human consumption," Journal of Marine Systems, vol. 75, pp. 305-313, 2009. View Article
[43] S. Lobban and P. J. Harrison, Seaweed Ecology and Physiology. Cambridge: Cambridge University Press, 1994.
[44] S. M. Coelho, J. W. Rijstenbil, and M. T. Brown, "Impacts of anthropogenic stresses on the early development stages of seaweeds," Journal of Aquatic Ecosystem Stress and Recovery, vol. 7, pp. 317-333, 2000. View Article
[45] G. B. Costa, M. R. L. de Felix, C. Simioni, F. Ramlov, E. R. Oliveira, D. T. Pereira, M. Maraschin, F. Chow, P. A. Horta, C. M. Lalau, C. H. da Costa, W. G. Matias, Z. L. Bouzon, and E. C. Schmidt, "Effects of copper and lead exposure on the ecophysiology of the brown seaweed Sargassum cymosum," Protoplasma, vol. 253, pp. 111-125, 2016. View Article
[46] S. Connan and D. B. Stengel, "Impacts of ambient salinity and copper on brown algae: 1. Interactive effects on photosynthesis, growth, and copper accumulation," Aquatic Toxicology, vol. 104, pp. 94-107, 2011. View Article
[47] H. Kupper, I. Setlik, M. Spiller, F. C. Kupper, and O. Prasil, "Heavy metal-induced inhibition of photosynthesis: Targets of in vivo heavy metal chlorophyll formation," Journal of Phycology, vol. 38, pp. 429-441, 2002. View Article
[48] P. R. Bond, M. T. Brown, R. M. Moate, M. Gledhill, S. J. Hill, and M. Nimmo, "Arrested development in Fucus spiralis (phaeophyceae) germlings exposed to copper," European Journal of Phycology, vol. 34, pp. 513-521, 1999. View Article
[49] J. G. Stewart, "Effects of lead on the growth of four species of red algae," Phycologia, vol. 16, pp. 31-36, 1977. View Article
[50] J. W. Markham, B. P. Kremer, and K. R. Sperling, "Effects of cadmium on Laminaria saccharina in culture," Marine Ecology Progress Series, vol. 3, pp. 31-39, 1980. View Article
[51] I. M. Munda and V. Hudnik, "The effects of Zn, Mn, and Co accumulation on growth and chemical composition of Fucus vesiculosus L. under different temperature and salinity conditions," Marine Ecology, vol. 9, pp. 213-225, 1988. View Article
[52] N. Favero and M. G. Frigo, "Biomonitoring of metal availability in the southern basin of the Lagoon of Venice (Italy) by means of macroalgae," Water Air and Soil Pollution, vol. 140, pp. 231-246, 2002. View Article
[53] J. C. Amiard, R. Journel, and H. Bacheley, "Influence of field and experimental exposure of mussels (Mytilus sp.) to nickel and vanadium on metallothionein concentration," Comparative Biochemistry and Physiology C: Toxicology & Pharmacology, vol. 147, pp. 378-385, 2008. View Article
[54] I. V. Seregin and V. B. Ivanov, "Physiological aspects of cadmium and lead toxic effects on higher plants," Russian Journal of Plant Physiology, vol. 48, pp. 523-544, 2001. View Article
[55] A. Serafim and M. J. Bebianno, "Effect of a polymetallic mixture on metal accumulation and metallothionein response in the clam Ruditapes decussatus," Aquatic Toxicology, vol. 99, pp. 370-378, 2010. View Article
[56] G. W. Bryan and L. G. Hummerstone, "Brown seaweed as an indicator of heavy metals in estuaries in Southwest England," Journal of the Marine Biological Association of the United Kingdom, vol. 53, pp. 705-720, 1973. View Article
[57] P. S. Rainbow, B. D. Smith, and S. S. S. Lau, "Biomonitoring of trace metal availabilities in the Thames estuary using a suite of littoral biomonitors," Journal of the Marine Biological Association of the United Kingdom, vol. 82, pp. 793-799, 2002. View Article
[58] G. W. Bryan, W. J. Langston, L. G. Hummerstone, and G. R. Burt, "A guide to the assessment of heavy metal contamination in estuaries," Occasional Publications. Marine Biological Association of the United Kingdom, vol. 4, pp. 1-92, 1985. View Article
[59] J. Collen, E. Pinto, M. Pedersen, and P. Colepicolo, "Induction of oxidative stress in the red macroalga Gracilaria tenuistipitata by pollutant metals," Archives of Environmental Contamination and Toxicology, vol. 45, pp. 337-342, 2003. View Article
[60] E. Pinto, T. C. S. Sigaud-Kutner, M. A. S. Leitao, O. K. Okamoto, D. Morse, and P. Colepicolo, "Heavy metal induced oxidative stress in algae," Journal of Phycology, vol. 39, pp. 1008-1018, 2003. View Article
[61] B. Szalontai, L. I. Horvath, M. Debreczeny, M. Droppa, and G. Horvath, "Molecular rearrangements of thylakoids after heavy metal poisoning, as seen by Fourier transform infrared (FTIR) and electron spin resonance (ESR) spectroscopy," Photosynthesis Research, vol. 61, pp. 241-252, 1999. View Article
[62] K. Haglund, M. Bjorklund, S. Gunnare, A. Sandberg, U. Olander, and M. Pedersen, "New method for toxicity assessment in marine and brackish environments using the macroalga Gracilaria tenuistipitata (Gracilariales, Rhodophyta)," Hydrobiologia, vol. 327, pp. 317-325, Jul 26 1996.
[63] D. J. Franklin, J. Humphreys, M. Harris, A. C. Jensen, R. J. H. Herbert, and D. A. Purdie, "An investigation into the annual cycle of phytoplankton abundance in Poole Harbour and its relationship with Manila clam nutrition," Report for the Marine Management Organisation, 2012.
[64] M. J. Bowes, D. V. Leach, and W. A. House, "Seasonal nutrient dynamics in a chalk stream: the River Frome, Dorset, UK," Science of the Total Environment, vol. 336, pp. 225-241, 2005. View Article
[65] K. A. Boyle, K. Kamer, and P. Fong, "Spatial and temporal patterns in sediment and water column nutrients in a eutrophic southern California estuary," Estuaries, vol. 27, pp. 378-388, 2004. View Article