Volume 2 - Year 2014 - Pages 59-65
DOI: 10.11159/ijepr.2014.009
Hybrid Clay Nanomaterials with Improved Affinity for Carbon Dioxide through Chemical Grafting of Amino Groups
Saadia Nousir, Andrei-Sergiu Sergentu¹, Tze Chieh Shiao, René Roy and Abdelkrim Azzouz*
Nanoqam, Department of Chemistry, University of Quebec in Montreal,
Montreal, Qc, Canada H3C3P8
¹ Laboratory of Catalysis and Microporous Materials (LCMM),
University of Bacau, Romania
azzouz.a@uqam.ca
Abstract - Chemical grafting of (3-aminopropyl) triethoxysilane on Na-montmorillonite in ethanol-water mixture or ethylene glycol as solvent resulted in two organoclays (NaMt-S-EW and NaMt-S-EG, respectively). The latter were characterized through X-ray diffraction, Fourier transform infrared spectroscopy, thermal analysis, differential scanning calorimetry and 29Si solid-state nuclear magnetic resonance (NMR). Nitrogen adsorption isotherms measurements revealed lower specific surface area as compared to the starting clay mineral. This was explained by the formation of compact lamella stacks bound by silylation at the edges of the clay sheets. Thermal programmed desorption analyses (TPD) revealed an improved affinity towards carbon dioxide (CO2) as compared to the starting clay mineral. NaMt-S-EW displayed higher affinity towards CO2 than NaMt-S-EG, with retention efficiency factor exceeding 16 µmol.m-2 for high amine content. Differential scanning calorimetry gave desorption enthalpy ranging from 148 to 467 kcal.mol-1, suggesting that only chemical interactions are involved between the amino groups grafted and CO2. CO2 retention capacity exceeding 1.0 mmol.g-1 with efficiency factor higher than 16 micromol.m-2 can be obtained for higher amine content, in optimum content, when no CO2 removal through forced convection takes place.
Keywords: Silylated montmorillonite, (3-aminopropyl)triethoxysilane, chemical grafting, TPD, CO2 retention.
© Copyright 2014 Authors - This is an Open Access article published under the Creative Commons Attribution License terms. Unrestricted use, distribution, and reproduction in any medium are permitted, provided the original work is properly cited.
Date Received: 2013-09-19
Date Accepted: 2014-02-23
Date Published: 2014-05-30
1. Introduction
A growing interest is now devoted to clay-based nanocomposites obtained through chemical grafting of organic silane moieties [1-4] and to the features of the silylating agents to be grafted [5,6]. Chemical grafting of amines has been intensively studied [7], but the use of clay minerals as inorganic supports for preparing CO2 adsorbents has been scarcely tackled. The solvent used during silylation is expected to play a significant role, because it should strongly influence the swelling capacity and dispersion grade of both the clay mineral lamellae and the chemical species to be grafted [8,9]. For instance, ethylene glycol (EG) displays a surface energy close to that of montmorillonite [10], and favor interaction between ethoxy-silanes and OH groups of the clay mineral.
Attempts to modify montmorillonite resulted in the insertion of a single molecular silane layer within the interlamellar space [7]. Ethanol/water mixtures were found to enhance hydrolysis and polymerization of the silane molecules into siloxane bonds [7,11,12]. These two processes can occcur even before clay pillaring [13], leading to the formation of a wide variety of polymer sizes and shapes. Reportedly, the amount of water in such reaction mixtures was found to play a key role [14]. After previous polymerization, if any, only those molecules having appropriate size and spatial geometry can be incorporated in the interlayer space. At the edges and the structural defects of montmorillonite layers, the terminal OH groups are expected to act as active sites for the silylation process [7,11]. Consequently, the grafting process of silane species should strongly depend on the surface density of the terminal OH groups. These sites should be relatively more accessible for silylation than those arising from structural defects, if any, or clay sheet edges sandwiched within the interlayer space. The accessibility to these potential interlayer silylation sites could be more or less accentuated by using different solvents that promote clay exfoliation.
For this purpose, the present study was achieved in order to clarify the role of the two solvents used, namely ethylene glycol and a water-ethanol mixture. The exfoliation grade of the solvent will be discussed in terms of specific surface area, porosity and CO2 retention capacity of the corresponding organoclay. The results obtained will certainly be very useful for designing effective silylation processes on lamellar crystalline matrices.
2. Experimental
2.1. Adsorbent PreparatioN
A montmorillonite-rich material ion-exchanged into the sodium form (NaMt) was used as inorganic support [15]. Further, NaMt-S samples were obtained through NaMt silylation with (3-aminopropyl)triethoxysilane (γ-APTES) according to two different grafting methods. The first one was carried out by impregnating 10 g NaMt under stirring at 80°C for 5h in 1000 mL of a 25:75 vol. water/ethanol mixture containing 3g of γ-APTES. The resulting powder (NaMt-S-EW-1, designated as NaMt-S-EW) was filtered, washed and dried at 80°C for 24 hours. Two other samples (NaMt-S-EW-2 and NaMt-S-EW-3) were prepared with [APTES:Montmorillonite] ratios of 0.8 and 1.0 g.g-1), respectively in the impregnating solution. The second method involved the preparation of a mixture containing on one hand a dispersion of 1g of NaMt in 100 mL of ethylene glycol (EG) under stirring, and a solution of 1g γ-APTES in 100 mL of ethylene glycol (EG). This mixture was stirred at room temperature for 30 min. The resulting product (NaMt-S-EG) was filtered and dried at 80 °C.
2.2. Characterization Thermal Desorption Measurements
X-Ray powder diffraction (XRD) was carried out by means of a Siemens D5000 instrument (Co-Kα at 1.7890 Å). BET measurements of the specific surface area and BJH assessment of porosity and pore size distribution were performed through nitrogen adsorption-desorption isotherm, using a Quantachrome device, with an Autosorb automated gas sorption system control. Thus, samples of 80-100 mg were previously dried at 80°C for 24 h, degassed at 80°C for 16 h under a 10-4 Torr vacuum and then the nitrogen adsorption was made at -195.7 °C. Thermal gravimetric analysis (TGA) was performed by means of a TG/TDA6200 thermal analyzer (Seiko Instrument Inc.) under a 120 mL.min−1 nitrogen stream and a 5°C.min−1 heating rate. The amount of the grafted APTES was assessed on the basis of the mass loss between 200 and 600°C. Besides, infrared spectra were recorded using a KBr IR cell and Fourier Transform Infrared spectroscopy equipment (Model IR 550, Magna Nicolet). 29
29Si nuclear magnetic-resonance (29Si NMR) analysis was achieved by means of a Brüker DSX-300 spectrometer operating at 12.5 KHz, at a contact time of 5 ms, a recycle delay of 1 s and a spinning rate of 12.5 KHz. Tetramethylsilane (TMS) was used as the external reference.
The CO2 retention capacity (CRC) of NaMt and NaMT-S samples was assessed by thermal programmed desorption of carbon dioxide (CO2-TPD) according to a procedure fully described elsewhere [16]. TPD measurements were performed between 20°C and 200°C under different dry nitrogen streams (1, 2, 5 and 15 mL.min-1) at a 5 °C/min heating rate, using a tubular glass reactor having a 10 mm internal diameter. The latter was incorporated in a tubular ceramic oven and coupled to a CO2-detector (Li-840A CO2/H2O Gas Analyzer). Prior to TPD investigations, CO2 was injected in excess at room temperature through the reactor under nitrogen flow rate (15 mL.min-1). Full saturation of the adsorbent and impregnation were achieved during fixed times according to the experiments, followed by a purge in order to release the excess CO2 not adsorbed at 20°C. Here, special measures were taken to avoid forced convection under strong nitrogen stream, which may affect CRC measurement accuracy [15,17].
3. Results and Discussion
3.1. Changes in the Surface Structure
FT-IR analyses revealed a shift of the Si-O stretching band from 1032 cm-1 for NaMt to 1038 cm-1 for both NaMt-S-EW-1 (designated as NaMt-S-EW) and NaMt-S-EG (Figure 1). This provides evidence of the strengthening of the Si-O bond belonging to the inorganic support, presumably due to the silylation process.
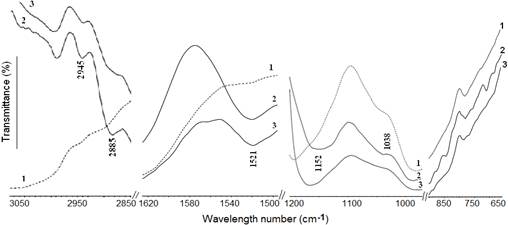
The presence of APTES on the clay mineral surface was supported by new bands observed at 1521, 2885 and 2945 cm−1, and assigned to the deformation of -CH2 and to both the asymmetric and symmetric stretching of the -CH belonging to the -CH2 groups respectively. Besides, other bands were also registered between 900-950 cm-1, and were attributed to the –NH2 bending.
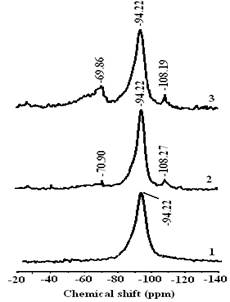
Amine grafting produced two additional 29Si signals at -70.9 and -108.27 ppm for NaMt-S-EG and at -69.86 and -108.19 ppm for NaMt-S-EW. These two signals, attributed to the hydrolyzed tridentate T3[Si(OSi)3R] (R = CH2CH2CH2NH2) and T4[Si(OSi)4, respectively indicate the formation of Clay-O-Si-R bonds, i.e. the chemical grafting of propylamine on the surface of montmorillonite.
3.2. Interlayer Structure and Changes Upon Silylation
NaMt-S-EW and NaMt-S-EG showed higher basal spacing of 1.67 and 1.89 nm respectively, as compared to NaMt (1.13 nm) (Figure 3). This indicates an increase in the interlayer space as a result of montmorillonite modification. The discrepancy between both basal spacing indicates a significant influence of the grafting procedure. Surprisingly, amine grafting seems to have a negative effect on the specific surface area, which dramatically decreased from 59 m2.g-1 for NaMt to 28 m2.g-1 (for NaMt-S-EG) and 21 m2.g-1 (for NaMt-S-EW) (Table 1). A possible explanation is that amine grafting through silylation on montmorillonite seems to promote mesoporosity (38 Å ≤ pore size ≤ 73 Å) at the expense of micropores. The latter almost totally disappeared by NaMt chemical modification. A deep analysis of the pore size distribution and XRD patterns provides arguments in this regard, inasmuch as NaMt-S-EG possesses a lower 001 spacing (1,89 nm) as compared to NaMt-S-EW (1,67 nm) (Figure 3) and a slightly higher pore volume (Figure 4).
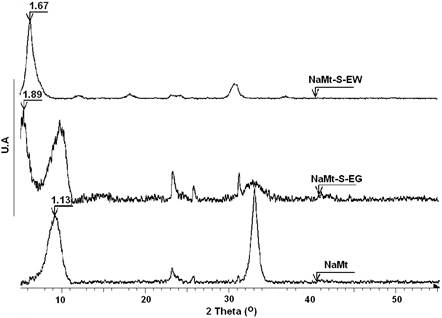
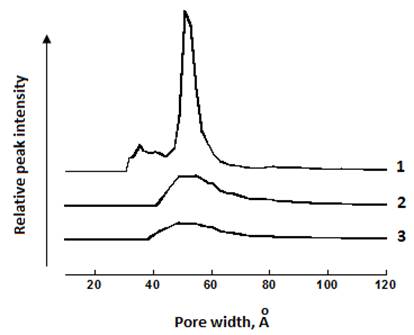
Nonetheless, the occurrence of two basal spacing for NaMt-S-EG suggests that a significant part of NaMt did not undergo full exfoliation. Here, amine grafting through silylation on the terminal OH groups located at the clay lamella edges is supposed to produce compact clay sheet stacks with low porosity and accessibility to the interlayer space [9]. Clay compaction, if any, should be more pronounced on NaMt-S-EG, presumably due to the strong sandwiching effect of ethylene glycol, well known to hinder clay delamination. In this case, the remaining part of mesopores accessible to CO2 molecules should arise only from the interparticle void volume. Subsequently, NaMt-S-EG is expected to retain less CO2 than NaMt-S-EW.
3.3. Thermal Stability
TG and DTG investigations of NaMt chemical modification showed the appearance of new mass loss peaks between 100 and 600°C, beside that of dehydration below 100°C and of dehydroxylation beyond 550°C belonging to montmorillonite (Figure 5 and Figure 6).
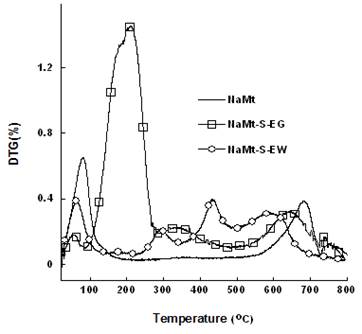
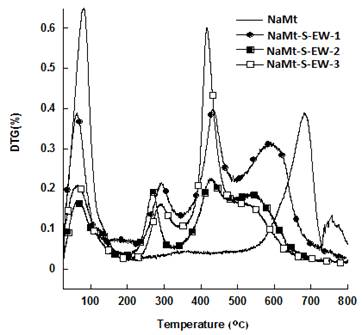
NaMt-S-EW samples were found to display higher thermal stability up to 250°C as compared to NaMt-S-EG, which starts decomposing even at 100°C. The mass losses registered beyond 250°C are common features of NaMt-S-EW samples. They must be due to the thermal decomposition of physically adsorbed APTES (330°C and 430°C) and chemically grafted amines (540°C). The latter showed increasing amount with increasing [APTES:Montmorillonite] ratio in the starting reaction mixture (0.3, 0.8 and 1.0 g.g-1) (Table 1).
Table 1. Some features of the as synthesized montmorillonite based materials.
Sample |
APTES contacted |
Mass Lossb |
-NH2 amount for different
number of silyl bridges |
CRCd |
SBET |
EFe |
|||
200-600°C |
490-600°C |
1 |
2 |
3 |
|||||
NaMt |
0 |
0.7 |
0.7 |
- |
- |
- |
1.25 |
59 |
0.021 |
NaMt-S-EG |
0.3 |
11.26 |
1.65 |
0.16 |
0.11 |
0.08 |
115 |
28 |
4.11 |
NaMt-S-EW-1f |
0.3 |
8.12 |
2.92 |
0.28 |
0.19 |
0.15 |
250 |
21 |
11.9 |
NaMt-S-EW-2 |
0.8 |
10.50 |
3.65 |
0.35 |
0.24 |
0.19 |
495 |
31.6 |
15.7 |
NaMt-S-EW-3 |
1.0 |
12.89 |
3.07 |
0.29 |
0.20 |
0.16 |
> 1000 |
31.2 |
> 32 |
aAmount of APTES in the impregnating solution contacted per gram of dry montmorillonite.
b Mass loss values measured through thermogravimetry between 200 and 600°C in air stream. This accounts for the amount of removed ethanol and propylamine, taking into account that the total decomposition of each APTES molecule results in one residual Si(OH)4 molecule deposited on the clay mineral surface.
c The number of amino groups grafted on montmorillonite was calculated for different number of silyl bridges by dividing the mass loss (g.g-1) by the sum of the molecular weights of propylamine (C2H7NH2, 59 g.mol-1) and of one (46 g.mol-1), two (2x46 g.mol-1) or three ethanol molecules (3x46 g.mol-1). These calculations take into account that one amino group may be grafted by APTES silylation with one, two or three clay silanol groups, resulting in the formation of one, two or three silyl bridges, respectively.
d The CRC value was expressed in terms of desorbed amount of CO2 between 20 and 80°C. These values were assessed by TPD (1.0 mL.min-1 nitrogen flow rate) after CO2 injection and purge at 15 mL.min-1 nitrogen throughput.
e The efficiency factor (EF) was defined as being the [CRC : specific surface area] ratio (Azzouz et al., 2013-a).
f Throughout the entire manuscript text, NaMt-S-EW-1 was designated as NaMt-S-EW.
The amount of amino groups incorporated assessed on the basis of the overall mass loss between 200 and 600°C gave [CO2:-NH2] mole ratios much lower than the expected 1:1 stoichiometric value, which is a special feature of the formation of a carbamate group from one amino group with one CO2 molecule. Any discrepancy from this value suggests that a part of amino groups do not contribute to CO2 retention, not being accessible, as previously stated.
In contrast, similar calculations on the basis of the third decomposition peak at 490-600°C provided values of the [CO2:-NH2] mole ratios close to unity, more particularly when assuming the formation of one silyl group via the loss of one ethanol molecule. Increasing number of hypothetical number of silyl groups up to three induced an increase of the [CO2:-NH2] mole ratio. This indicates that an excess of CO2 with respect to stoichiometry adsorbs also via physical interactions, presumably due to the base character induced in the adsorbent bulk.
3.4. Affinity towards CO2 and Heat of CO2 Retention
NaMt-S-EW showed higher amount of thermally desorbed CO2 as compared to NaMt-S-EG and the starting NaMt material (Figure 7). The thermal stability of both adsorbents was confirmed by reproducible CO2-TPD triplicate performed between 20 and 200°C for NaMt-S-EW and between 20 and 80°C for NaMt-S-EG under constant moisture content (Figure 8 and 9). Dehydration after repetitive adsorption-desorption cycles was found to reduce unavoidably the amount of desorbed CO2. This was already explained by the contribution of water in CO2 retention [17,18].
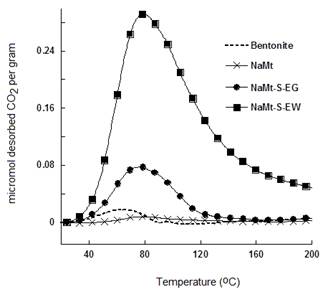
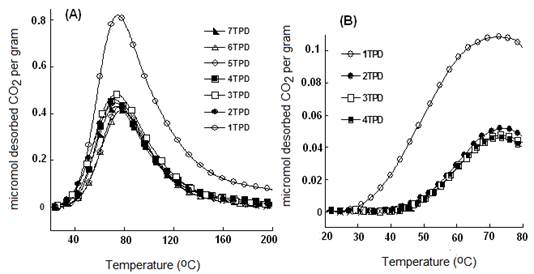
By increasing the nitrogen flow rates from 1 to 15 mL.min-1, the amount of desorbed CO2 decreased dramatically from ca. 70 to ca. 12-15 µmol.g-1 for NaMt-S-EW, and from 25-35 to less than 5 µmol.g-1 for NaMt-S-EG (Figure 10). Here also, strong nitrogen stream are supposed to remove CO2 even at room temperature through forced convection, as already reported for OH-enriched montmorillonites [15,17]. This result is of great importance, because it demonstrates that CO2 removal without heating is possible even when chemical adsorption is involved.
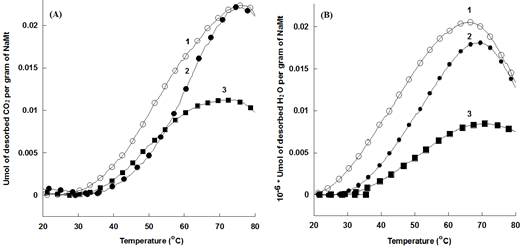
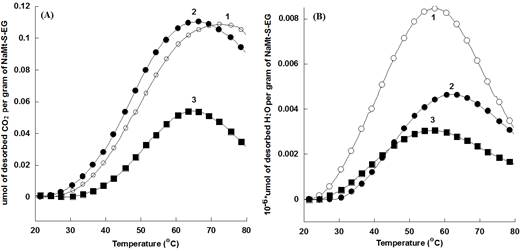
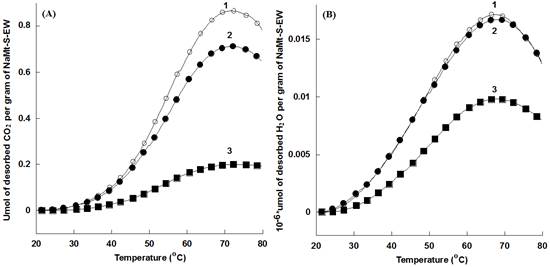
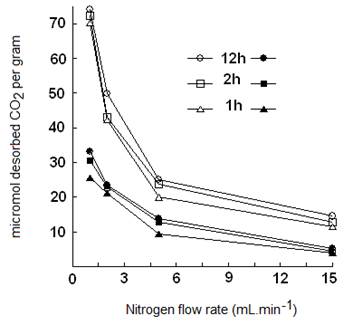
NaMt-S-EG: (dark symbols); NaMt-S-EW (white symbols). Saturation of both samples with CO2 was performed in static mode (without nitrogen stream), and was followed by a purge at 20°C in a 15 mL.min-1 dry nitrogen stream till no CO2 is detected. TPD experiments were performed at a heating rate of 5 °C/min up to the upper temperature value, which was then maintained constant for 20 min.
DSC measurements revealed that CO2 desorption requires heat amount of 264-467 kcal.mol-1 for NaMt-S-EW between 26 and 153°C, and of 148-229 kcal.mol-1 for NaMt-S-EG between 26 and 115°C. This indicates that CO2 adsorption involves stronger interaction with NaMt-S-EW as compared to NaMt-S-EG, and provides clear evidence that only chemical interaction is involved between CO2 and the amino groups incorporated. Under optimum conditions, i.e. when CO2 removal by forced convection at room temperature is avoided or minimized, the CRC value may exceed by far 1.0 mmol.g-1 (Table 1).
3.5. Effect of Grafting Grade
These values explain the relatively high affinity of NaMt-S-EW towards CO2 as compared to NaMt-S-EG, in agreement with its higher amine content. Deeper insights in this regard showed that the amount of CO2 thermally desorbed by TPD increases with increasing amount of injected CO2 (Figure 11).
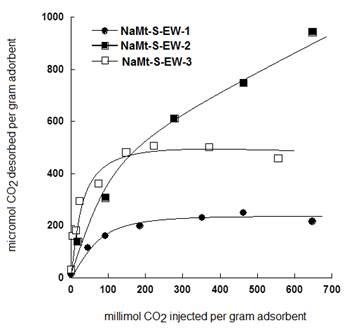
Saturation was attained at 350 mmol.g-1 for NaMt-S-EW-1 and 200 mmol.g-1 for NaMt-S-EW-2, providing CO2 retention capacity (CRC) of 240-250 mmol.g-1 and 490-500 mmol.g-1, respectively. This suggests an almost linear proportionality with respect to the amount of incorporated amine. No saturation took place on NaMt-S-EW-3, even up to 700 mmol.g-1 of injected CO2. This indicates CRC values much higher 1000 mmol.g-1, in agreement with other data [19].
Indeed, in spite of its lower specific surface area, NaMt-S-EW showed an efficiency factor (11.9) approximately three times higher than that of NaMt-S-EG (4.11). As compared to OH-enriched montmorillonite [15,17], NaMt-S-EW showed appreciable efficiency factor that may exceed 16 micromol CO2.m-2 for higher amine content.
4. Conclusion
Grafting montmorillonite with (3-aminopropyl)triethoxysilane induced a significant improvement of the CO2 retention capacity as compared to the starting clay mineral. The latter was found to be influenced by the grafting procedure. It was found that the use of ethanol/water mixture in the preparation leads to materials with high thermal stability and affinity towards CO2. NaMt-S-EW displayed higher affinity towards CO2 than NaMt-S-EG, with retention efficiency factor exceeding 16 µmol.m-2 for high amine content. Differential scanning calorimetry demonstrated that only chemical interactions are involved between the amino groups grafted and CO2. However, CO2 removal without heating is possible upon mere forced convection at high nitrogen throughput. This innovative concept opens new prospects for providing new inorganic–organic materials that act as respiratory matrices for the reversible capture of polluting gases and other environmental purposes.
Acknowledgements
This work was supported by a grant from MDEIE-FQRNT to R.R. and A.A.
References
[1] K.W. Park, S.Y. Jeong, O.Y. Kwon "Interlamellar silylation of H-kenyaite with 3-aminopropyltriethoxysilane" Applied clay science, 27(1-2), 2004, pp. 21-27. View Article
[2] N.N. Herrera, J.M. Letoffe, J.L. Putaux "Aqueous dispersions of silane-functionalized laponite clay platelets: A first step toward the elaboration of water-based polymer/clay nanocomposites", Langmuir, 20(5), 2004, pp. 1564-1571. View Article
[3] M. Park, I.K. Shim, E.Y. Jung "Modification of external surface of laponite by silane grafting", Journal of Physics and Chemistry Solids, 65(2-3), 2004, pp. 499-501. View Article
[4] K. Isoda, K. Kuroda "Interlamellar grafting of γ-meththacryloxypro- pylsilyl groups on magadiite and copolymerization with methyl methacrylate", Chemistry of Materials, 12, 2000, pp. 1702-1707. View Article
[5] J.X. Zhu, H.P. He, J.G. Guo, D. Yang, X.D. Xie "Arrangement models of alkylammonium cations in the interlayer of HDTMA+ pillared montmorillonites", Chinese Science Bulletin, 48(4), 2003, pp. 368-372. View Article
[6] K.A. Carrado, L.Q. Xu, R. Csencsits, J.V. Muntean "Use of organo- and alkoxysilanes in the synthesis of grafted and pristine clays" Chemistry of Materials, 13(10), 2001, pp. 3766-3773. View Article
[7] H.P. He, J. Duchet, J. Galy, J.F. Gerard "Grafting of swelling clay materials with 3-aminopropyltriethoxysilane" Journal of Colloid and Interface Science, 288(1), 2005, pp. 171-176. View Article
[8] S. Linna, Q. Tao, H. He, J. Zhu, P. Yuan, R. Zhu "Silylation of montmorillonite surfaces: Dependence on solvent nature" Journal of Colloid and Interface Science, 377(1), 2012, pp. 328-333. View Article
[9] A.M. Shanmugharaj, K.Y. Rhee, S.H. Ryu "Influence of dispersing medium on grafting of aminopropyltriethoxysilane in swelling clay materials" Journal of Colloid and Interface Science, 298(2), 2006, pp. 854-859. View Article
[10] J.E. Jordan "Organophilic bentonites I. Swelling in organic liquids" Journal of Physical and Colloid Chemistry, 53(2), 1949, pp. 294-306. View Article
[11] S. Ek, E.I. Iiskola, L. Niinistö "Atomic layer deposition of aminofunctionalized silica surfaces using N-(2-Aminoethyl)-3-aminopropyltrimethoxysilane as a silylating agent" The Journal of Physical Chemistry B, 108(28), 2004, pp. 9650-9655. View Article
[12] C.J. Brinker, G.W. Scherer "Sol-Gel Science, the Physics and Chemistry of Sol-Gel Processing" Academic Press, London, 1990 pp. 97. View Article
[13] J. Ahenach, P. Cool, E. Vansant, O. Lebedev, J.V. Landuyt "Influence of water on the pillaring of montmorillonite with aminopropyltriethoxysilane" Physical Chemistry Chemical Physics, 1(15), 1999, pp. 3703-3708. View Article
[14] M.M. Sprung, F.O. Guenther "The partial hydrolysis of methyltriethoxysilane" Journal of the American. Chemistry Society, 77(15), 1955, pp. 3990-3996. View Article
[15] A. Azzouz, N. Platon, S. Nousir, K. Ghomari, D. Nistor, T.C. Shiao, R. Roy "OH-enriched organo-montmorillonites for potential applications in carbon dioxide separation and concentration" Separation and Purification Technology, 108, 2013, pp. 181-188. View Article
[16] A. Azzouz, D. Nistor, D. Miron, A.V. Ursu, T. Sajin, F. Monette, P. Niquette, R. Hausler "Assessment of acid–base strength distribution of ion-exchanged montmorillonites through NH3 and CO2-TPD measurements" Thermochimica Acta, 449(1-2), 2006, pp. 27-34. View Article
[17] S. Nousir, N. Platon, K. Ghomari, A.S. Sergentu, T.C. Shiao, G. Hersant, J.Y. Bergeron, R. Roy, A. Azzouz "Correlation between the hydrophilic character and affinity towards carbon dioxide of montmorillonite-supported polyalcohols" Journal of Colloid and Interface Science, 402, 2013, PP. 215-222. View Article
[18] A. Azzouz, S. Nousir, N. Platon, K. Ghomari, T.C. Shiao, G. Hersant, J.Y. Bergeron, R. Roy "Truly reversible capture of CO2 by montmorillonite intercalated with soya oil-derived polyglycerols" International Journal of Greenhouse Gas Control, 17, 2013, pp. 140–147. View Article
[19] M.L. Gray, Y. Soong, K.J. Champagne, R.W. Stevens Jr., P. Toochinda, S.S.C. Chuang "Solid amine CO2 capture sorbents" Fuel Chemistry Division Preprints, 47(1), (2002). pp. 63-64. View Article