Volume 1 - Year 2012 - Pages 98-103
DOI: 10.11159/ijepr.2012.014
Effects of Hydrolysis and Torrefaction on Pyrolysis Product Distribution of Spent Mushroom Compost (SMC)
Rene Garrido, Maria Nydia Ruiz-Felix, Justinus A. Satrio
Department of Chemical Engineering, Villanova University
800 East Lancaster Avenue, Villanova PA 19085
ren.garrido@villanova.edu; mruizfel@villanova.edu; justinus.satrio@villanova.edu
Abstract - This study investigates the use of spent mushroom compost (SMC) as a potential feedstock for the production of bio-oil via fast pyrolysis process. It was found that, although SMC can be converted to bio-oil, due to its high moisture and ash contents, fast pyrolysis of SMC results in low yield and quality of bio-oil. Reduction of the moisture and ash contents in SMC is the key element to make SMC a desirable feedstock for biomass conversion. This investigation has revealed that pyrolysis of SMC that has been treated by a combination of hydrolysis and torrefaction processes results in a higher yield of bio-oil production. The biomass that was only pretreated with torrefaction produces a lower yield of bio-oil compared to those that have been hydrolyzed or hydrolyzed then torrefied.
Keywords: SMC, Biomass Conversion, Acid Hydrolysis, Torrefaction, Pyrolysis
© Copyright 2012 Authors - This is an Open Access article published under the Creative Commons Attribution License terms. Unrestricted use, distribution, and reproduction in any medium are permitted, provided the original work is properly cited.
1. Introduction
Biomass is a renewable resource that can be converted to fuels and chemical products via biological, thermochemical processes and their combinations. Although fossil fuels, such as petroleum oil and coal, have their foundation in ancient biomass, they have been "out" of the carbon cycle for a long time. Their combustion consequently disturbs the carbon dioxide content in the atmosphere. Biomass, specifically lignocellulosic biomass, as the only carbon-containing renewable energy sources can be classified as natural (without human intervention), residuals (by-product/waste generated by agricultural activities, forestry, and industry wood processing), and energy crops (for the production of biofuels). The complex nature of lignocellulosic biomass poses a substantial challenge to the large-scale biomass utilization. Lignocellulosic materials contain highly valuable carbohydrates (cellulose and hemicelluloses) and lignin, which have different chemical structures and properties. The carbohydrate (sugar) polymers are tightly bound to the lignin, which makes utilizing the individual components of biomass separately challenging. Various biochemical and thermochemical processes, including their combination, are currently being explored by researchers in research institutions, academia and industry for converting numerous types of biomass materials. While it is technologically viable to exploit lignocellulosic materials and organic wastes into energy, chemicals and fuels, the cost related with it needs to be lowered. It also must be demonstrated that a commercial scale biomass utilization process is environmentally sound, and there is a need to find an inexpensive and widely available lignocellulosic source of biomass (Teymouri et al., 2005). The focus of this study is to explore the potential of spent mushroom compost (SMC) as a source of bioenergy.
1.1 Spent Mushroom Compost as Bio-energy Source
In term of logistics, ideal biomass materials are those that are readily available for continuous supplies in large quantities without having to collect and transport them in a large area. Kennett Square, a 1.1 square-mile borough in Chester County, Pennsylvania and located approximately 30 miles southwest of Villanova University, is known as the mushroom capital of the world (Mushroom Festival Inc. , 2012). The borough is home to enough mushroom farming to produce over a million pounds of mushrooms weekly. Similar to any production/processing facilities, mushroom production industries have their share of inputs and outputs. When discussing the sustainability of the mushroom production processes, it is important to realize what each of these inputs and outputs are.
The life cycle of the various types of mushroom fungi are similar. There is a substrate of decomposing organic matter that gets inoculated by the mushroom spores. These spores grow into filaments called hyphae and spread throughout the substrate. It is at this stage that the mushrooms assist in decomposition as they turn the organic matter into usable food for continued growth.
One of the most important considerations when dealing with mushroom growing is the solid waste product, called spent mushroom compost or SMC. Spent mushroom compost (SMC) is the remains of the compost in which mushrooms are produced (Williams et al., 2001). SMC consists of a combination of initial substrate and the casing layer after all nutrients have been exhausted from the substrate. It is known that each mushroom farm produces 60 to 70 thousand tons of SMC each year. In Kennett Square, currently there are 47 mushroom growers. From these mushroom farms, hundreds of thousands of tons of SMC are produced each year. Since it is unsustainable and harmful to the environment to store SMC without care (Finney et al., 2009), SMC must be disposed of either by a contractor or by other means (Williams et al., 2001). Currently, the majority of the SMC is either spread on farm land as fertilizer or discarded with a small portion being use in horticulture (Williams et al., 2001).
The continuous availability of SMC in large quantities in Kennet Square makes SMC an attractive resource in terms of logistics (i.e. collection and transportation). However, the low quality, particularly low heating value, of SMC due to its high moisture and ash content, makes SMC, used as it is, a less than ideal bioenergy feedstock. Lignocellulosic recalcitrance is one of the primary impediments in the successful implementation of waste biomass as an effective feedstock (Balan et al., 2007). Pre-treatment of biomass to reduce this intrinsic recalcitrance is critical to help improve bioconversion. SMC is a renewable source that needs treatment to improve its quality as bioenergy feedstock.
At Villanova University, it is proposed to utilize SMC to produce fuels and chemical products by using a combination of pre-treatment processes, biochemical conversion process (fermentation) and thermochemical process (fast pyrolysis) as shown in Fig. 1.
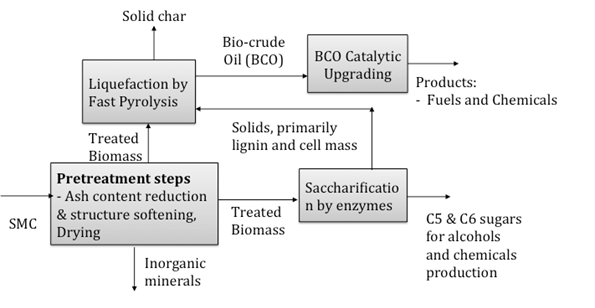
This paper reports a study on the effects of acid hydrolysis and torrefaction as pre-treatment processes of SMC before the material undergoes fast pyrolysis. This involves assessing optimum conditions for acid concentration (H3PO4) and torrefaction temperatures to determine its suitability to reduce ash and moisture content before pyrolysis.
2. Material and Methods
2.1 Feedstock
Spent mushroom compost (SMC) was used in this experiment as the raw lignocellulosic biomass. Samples of SMC were collected from the Modern Farm mushrooms (Avondale, PA 19311). All samples were dried at 105°C and carefully kept in cool storage until use to prevent decomposition. Several key physicochemical properties of SMC, provided by the Modern Mushroom Farms, are presented in Table 1. For preliminary analysis, a portion of the dried SMC materials was ground and screened using a 40 mesh screen size for preliminary analysis.
Table 1. Preliminary chemical composition of SMC.
Value |
||
Properties |
As received wt% |
Moisture free wt% |
Proximate Analysis |
||
Moisture |
56.24 |
- |
Ash |
9.82 |
22.43 |
Volatile Matter |
28.52 |
65.18 |
Fixed Carbon |
5.42 |
12.39 |
Total |
100.00 |
100.00 |
Ultimate Analysis |
||
Moisture |
56.24 |
- |
Hydrogen |
1.49 |
3.40 |
Carbon |
17.82 |
40.73 |
Nitrogen |
1.89 |
4.31 |
Sulphur |
0.93 |
2.12 |
Oxygen |
11.81 |
26.99 |
Ash |
9.82 |
22.43 |
Total |
100.00 |
100.00 |
Heating Value |
||
HHV [BTU/lb] |
2803 |
6405 |
2.2 Pre-treatments
Pretreatment is the one of the most costly steps in the conversion of lignocellulose, accounting for about 33% of the total processing cost. (Brown, 2003).
Two pre-treatment processes were considered to lessen some of the recalcitrant properties of SMC. The pretreatments selected were acid hydrolysis and torrefaction, which function to reduce the ash content and moisture, respectively.
2.2.1 Acid Hydrolysis
Three basic methods for hydrolyzing lignocellulosic materials are available: concentrated-acid hydrolysis, dilute-acid hydrolysis, and enzymatic hydrolysis. (Brown, 2003).
Dilute acid hydrolysis is an effective biomass pretreatment. This process modifies the structure of the biomass by breaking/loosening the lignin, solubilizing hemicellulose, and disrupting the crystalline structure of cellulose (Mosier et al., 2005). During hydrolysis, water is added to xylan molecules forming xylose. Further degradation of xylose forms furfural (Jensen et al., 2008). A major challenge in acid hydrolysis is the selection of acid and its concentration of the acid that is used to give optimal results. Too high acid concentration can degrade the glucose molecules into less desired compounds (Xiang et al., 2004). The solid residues resulting from the acid hydrolysis can total up to 50% wt. of the biomass materials. To obtain the maximum efficiency from the treated biomass, the solid residue can be used as feedstock for other conversion process, for instance fast pyrolysis. Fast pyrolysis of the solid residues produces primarily a liquid product, known as bio-oil. By-products of fast pyrolysis are a solid product, known as biochar, and a mixture of non-condensable gases (Melligan et al., 2012).
Various acids have been used for hydrolysing biomass. Sulphuric acid and hydrochloric acid are two acids that have been reported for the acid hydrolysis of lignocellulosic materials (Gamez et al., 2006) (Yat et al., 2008). The use of these aggressive acids causes the glucose to degrade, producing furfural or acetic acid (Romero et al., 2007). Therefore, the research of less destructive acid is necessary. It has been reported that phosphoric acid is one possible substitution. Romero et al, (2007) proved that the use of phosphoric acid decreases the production of furfural and acetic acid in the treatment of olive tree pruning and potato peels. Furthermore, phosphoric acid increases the conversion of hemicellulose up to 77% when a concentration of 8% wt. of acid is used, and up to 82.5% when a concentration of 2.5% wt. of acid is used. (Romero et al., 2007) (Lenihan et al., 2012)
2.2.2 Torrefaction
Lignocellulosic biomass, as it is, is typically not ideal to be utilized as feedstock. Typically lignocellulosic biomass has a high moisture content, low bulk density, low energy density, and degrades during storage (Medic et al., 2012). These properties can be modified using torrefaction as a pre-treatment process. Torrefaction is a thermochemical process, i.e. a mild pyrolysis, which takes place at temperatures ranging from 200°C to 300°C. (Wang et al., 2006) (Bourgeois et al., 1984) (Prins et al., 2006). Depending on process temperature during torrefaction process, hemicellulose is decomposed to volatiles and non-condensable gases (Gaur et al., 1998), (Prins et al., 2006). After torrefaction, three products are obtained: a solid product, an acidic aqueous liquid product, and a mixture of non-condensable (carbon monoxide, carbon dioxide, hydrogen, and methane). The solid product is expected to have very low moisture content, higher heating value, and is easier to grind (Bergman, 2004). If torrefaction is included as a pre-treatment for the biomass during the biofuel process, the quality and efficiency of biofuels can be increased.
In this study, torrefaction of the SMC was performed by using a 2.635 in (6.7 cm)-ID tubular reactor which is heated by a BlueM tubular furnace. The length of the heated portion of the tube is 24 in (61 cm). The tubular reactor has a gas inlet and an outlet. The outlet line is equipped with a condenser system to collect the liquid product and is connected to an infrared-type continuous gas analyser manufactured by Dejaye Technologies to measure the non-condensable gas concentrations in the outlet stream after it passes the condenser system. In a typical experiment, torrefaction was performed using 20 grams of biomass. The biomass sample was placed in a small wire-mesh basket which was inserted to the center of the tubular reactor after the reactor was heated to the desired temperature. Nitrogen was used to purge the reactor and then kept at a rate of 80ml/min before the experiment started. Once the biomass was placed inside the reactor, the experiment was set to run for 30 minutes. At the completion of the torrefaction, the furnace was turned off, the biomass sample was pulled out from the reactor heating zone and then taken out from the reactor after the reactor was cooled down to close to the room temperature.
2.3 Pyrolyzer-GC-MS Experiments
Fast pyrolysis experiments were performed to evaluate the effects of acid hydrolysis and torrefaction of SMC on the fast pyrolysis product yields and the distribution of the chemical constituents in the bio-oil product. The experiments were performed at 500°C under an inert atmosphere using a micropyrolyzer (model 5200, CDS Analytical, Inc.). The micro-pyrolysis unit involved a quartz pyrolysis tube that can be preheated to a desired temperature (500°C in our study) with a furnace, an interface and a column adapter tube with needle (inserted into the gas chromatograph (GC) injector). The loaded quartz tubes fell freely into the preheated furnace by gravity in a short time period during which the sample was heated to the pyrolysis temperature, ensuring rapid pyrolysis (20°C/ms). The pyrolysis vapors were directly swept into the GC (HP 5890 Series II plus) using helium as the carrier gas. The constituents of the pyrolysis vapor were separated in the GC column and identified using a mass spectrometer (MS HP 5972 series). The method of chromatographic separation of pyrolysis products was adapted from Patwardhan, et al. 2009. In the method, the injector temperature program of 300 °C and a gas split ratio 1:100 were used. The GC oven temperature began with a 3 min. hold at 35°C followed by heating to 130°C at 3 °C/min and then 300°C at 7°C/min. To evaluate the mass balance of fast pyrolysis process, samples were weighed before and after pyrolysis using a Sartorius MC5 microbalance (5.1 g capacity and readability of 1 µg) to determine the initial sample weight and the weight of the final residual char.
Table 2. Proximate and Ultimate analysis of SMC.
|
Pyrolyzed |
||||||
|
SMC |
SMCH |
SMCT |
SMCH/T |
SMCH |
SMCT |
SMCH/T |
Properties |
|||||||
Proximate Analysis |
|||||||
Moisture |
64.35% |
9.56% |
2.58% |
4.02% |
- |
- |
- |
Volatile Matter* |
37.68% |
63.73% |
38.57% |
61.42% |
30.94% |
0.92% |
32.34% |
Fixed Carbon* |
28.85% |
25.09% |
29.62% |
11.90% |
19.62% |
46.71% |
16.97% |
Ash* |
33.47% |
11.18% |
31.81% |
26.69% |
49.44% |
52.37% |
50.69% |
Ultimate Analysis |
|||||||
Carbon |
35.52% |
44.98% |
36.42% |
35.53% |
26.56% |
30.17% |
25.51% |
Hydrogen |
3.84% |
5.26% |
3.93% |
4.43% |
2.94% |
2.49% |
2.89% |
Oxygen |
26.71% |
37.96% |
27.36% |
32.85% |
20.67% |
14.64% |
20.57% |
Ash |
33.47% |
11.18% |
31.81% |
26.69% |
49.44% |
52.37% |
50.69% |
H: hydrolysed, T: torrefied, H/T: hydrolysed and then torrefied
* Composition calculated on a dry basis.
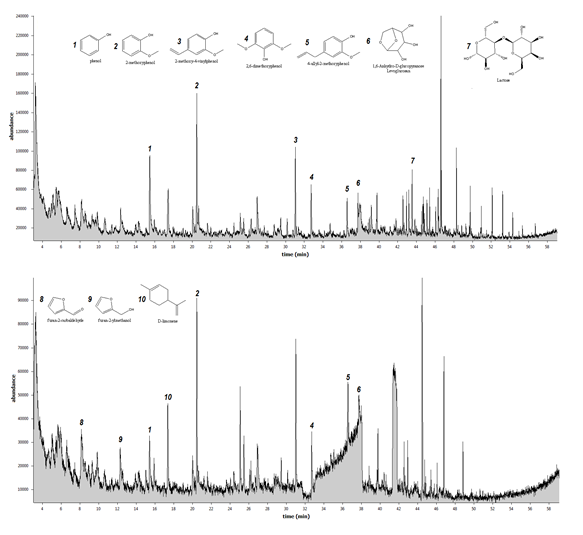
3. Results and Discussions
To properly determine the effects on pyrolyzed SMC, samples were either hydrolyzed or torrefied separately, or treated by a combination of hydrolysis and torrefaction before pyrolysis.
Also, to validate the results from each pretreatment and pyrolysis, samples were mixed uniformly and done in triplicates for statistical value.
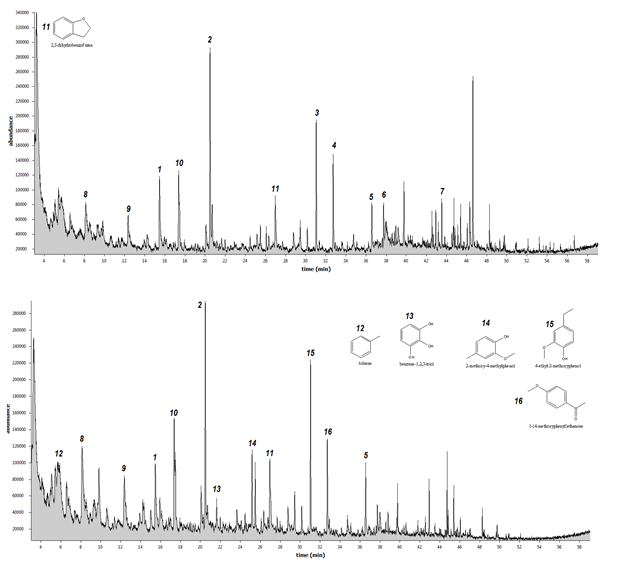
The samples were categorized as SMCH, SMCT and SMCH/T, respectively, according to the pre-treatment process. The hydrolysis was performed at 100oC with only water. The torrefaction was carried out at 200oC for 30 minutes, and the pyrolysis residence time was 10 seconds with a temperature of 500oC. To determine how the pretreatments affect the biomass recalcitrant, proximate analysis was performed to determine moisture content, volatile matter, fixed carbon and ash (Donahue et al., 2009)
Thermogravimetric analysis (TGA) is a high precision method for the study of pyrolysis at low heating rates; it can provide information about the partial processes and reaction kinetics (Varhegyi et al., 2011).
Although TGA has been frequently employed in the kinetic modeling of the thermal degradation of biomass materials, Parikh et al (2007) determined a way to link the proximate analysis from TGA to estimate the content carbon, oxygen and hydrogen from ultimate analysis by using empirical correlations. Results of the proximate analysis using TGA and the ultimate analysis using the correlation method developed by Parikh et al (2007) are shown in Table From Table 2, all the calculations were made based on the solid biomass remaining where the first three columns are the samples before pyrolysis, and the last three columns represent the pyrolyzed solid samples. There is a dramatic decrease of ash content in the hydrolysed samples versus a dramatic decrease of volatile matter on torrefied samples. The combined pre-treatment presents a milder decrease on the water and ash content of biomass.
Fig. 2 and Fig. 3 show the product distribution of fast pyrolysis of dried SCM, hydrolysed SMC (SMCH), torrefied SMC (SMCT), and hydrolysed/torrefied SMC (SMCH/T). It can be seen that pre-treatment of SMC by hydrolysis, torrefaction, or its combination affects the product distribution of the fast pyrolysis. The components of fast pyrolysis are compounds primarily derived from carbohydrates and lignin decomposition. It is interesting to observe that the abundance level of levoglucosan in torrefied SMC (fig. 3 top) is comparatively higher than those from untreated SMC (fig. 2 top), and not present at all in hydrolysed SMC (fig. 2 bottom). Levoglucosan is a sugar compound in bio-oil, which is an important building block for the production of various chemical products. (Bennett et al., 2009)
Another chemical worthwhile observing is lactose, which is a disaccharide derived from the condensation of glucose and lactose. It was observed that lactose only presents in the bio-oil product from the untreated SMC and not from the pre-treated SMC samples. One of the reasons is that a torrefaction temperature causes the biomass to break down its fibrous structure, particularly hemicellulose and lactose is one of the products derived from hemicellulose.
4. Conclusions
Spent mushroom composts (SMC), which is the waste product from mushroom producing farm, has the potential to be used as a renewable feedstock for the production of fuels and chemical products. The high moisture and ash content of SMC makes the renewable material not ideal to be used as it is. From this study, pretreatments of SMC by hydrolysis, torrefaction, and their combination process, have the potential to improve the quality of SMC as biomass feedstock. These pretreatment processes alter the distribution of the chemical constituents in the fast pyrolysis products. Further study on the effects of the acid concentration levels during hydrolysis is needed to determine optimum conditions along with different torrefaction temperatures.
5. Future Work
Currently the Biomass Resources and Conversion Technologies (BRCT) laboratory of Villanova University is working on establishing the optimum condition for torrefaction temperatures and acid hydrolysis concentrations for improving the quality of SMC as fast pyrolysis feedstock. More work is needed to explore further how to decrease the ash concentration in SMC and recover ash as possible valuable by-product.
6. Acknowledgements
Funding to support this research work partially comes from the Keystone Innovation Started Kit (KISK) grant from Pennsylvania's Department of Community and Economic Development. The authors would like to acknowledge Modern Mushroom Farms in Avondale, Pennsylvania, particularly the farm manager, Mr. Gene Taylor, for providing the spent mushroom compost samples and the proximate analysis information. Finally, the authors also would like to acknowledge the following Villanova University former graduate students: Justin Yeash for initiating the spent mushroom compost research at VU and Trevor Misera for designing and constructing the tubular reactor system used for the SMC study, and the following undergraduate researchers for their assistance in the laboratory: Richard Adrian, Lindsay Peterson and Joseph Reckamp.
References
Balan, V., da Costa Sousa, L., Chundawat, S. P. S., Vismeh, R., Jones, A. D., Dale, B. E. (2007)Mushroom spent straw: a potential substrate for an ethanol-based biorefinery. Journal of Industrial Microbiology & Biotechnology 35, 293 - 301. View Article
Bennett, N. M., Helle, S. S., Duff, S. J. B (2009) Extraction and hydrolysis od levoglucosan from pyrolysis oil. Bioresource Technology 100, 23 6059-6063. View Article
Bergman, P., Boersma, J., Kiel, J., Prins, M., Ptanski, K., Janssen, F. (2004) Proceedings of the second world conference on biomass for energy, industry and climate protection. Rome. View Article
Bourgeois, J. P., J. Doat, H. Egneus, and A. Ellegard. (1984) Biomass conversion. Elsevier Applied Sciences.
Brown, C. R. (2003) Biorenewable Resources. Engineering New Products from Agriculture. Iowa: Blackwell Publishing.
Donahue, C. J., Rais, E. A. (2009) Proximate Analysis of Coal. Journal of Chemical Education 86, 2 222 - 224. View Article
Finney, K. N., Sharifi, V. N., Swithenbank, J. (2009) Combustion of spent mushroom compost and coal tailing pellets in a fluidised-bed. Renewable Energy 34, 3, 860 - 868. View Article
Gámez, S., Gonzálex-Cabriales, J. J., Ramírez, J. A., Garrote, G., Vázquex, M. (2006) Study of the hydrolysis of sugar cane bagasse using phosphoric acid. Journal of Food Engineering 74, 1 78 - 88. View Article
Gaur, S., Reed, T. (1998) Thermal data for natural and synthetic fuels. New York: Marcel Dekker. View Article
Jensen, J., Morinelly J., Aglan A., Mix A., Shonnard, D. (2008) Kinetic characterization of biomass dilute sulfiric acid hydrolysis: mixtures of hardwoods, softwood, ans switchgrass. AIChE Journal 54, 6, 1637 - 1645. View Article
Lenihan, P., Orozco, A., O'Neill, E., Ahmad, M. N. M., Rooney, D. W., Mangwandi, C., Walker, G. M. (2012) Kinetic modelling of dilute Acid Hydrolysis of Lignocellulosic Biomas. Vol. 156, in Biofuel Production - Recent Developments and Prospects, 293 - 308. Chemical Engineering Journal, 2012. View Article
Medic, D., Darr, M., Shah, A., Potter, B., Zimmerman, J. (2012) Effects of torrefaction process parameters on biomass feedstock upgrading. Fuel 91, 1 147 - 154. View Article
Melligan, F., Dussan, K., Auccaise, R., Novotny, E. H., Leahy, J. J., Hayes, M. H. B., Kwapinski, W. Characterisation of the products from pyrolysis of residues after acid hydrolysis of Miscanthus. Bioresource Technology 108 (2012): 258 - 263. View Article
Moser, Evan. (2008) Making a better feedstock by torrefaction and the removal of Ash. B.Sc. Thesis, Iowa : Iowa State University.
Mosier, N., Wyman, C., Dale, B., Elander, R., Lee, Y. Y., Holtzapple, M., Ladisch, M. (2005) Features of promising technologies for pretreatment of lignocellulosic biomass. Bioresource Technology 96,6 ,673 - 686. View Article
Parikh, J., Channiwala, S. A., Ghosal, G. K. (2007) A correlation for calculating elemental composition from proximate analysis of biomass materials. Fuel 86, 1710 - 1719. View Article
Patwardhan, P. R., Satrio, J. A., Brown, R. C., Shanks, B. H., (2009) Product distribution from fast pyrolysis of glucose-based carbohydrates. Journal of Analytical and Applied Pyrolysis 86, 2, 323 - 330. View Article
Prins, M. J., Ptasinski, K. J., Janssen, F. J. J. G. (2006) Torrefaction of wood. Part 2. Analysis of products. Journal of Analytical and Applied Pyrolysis 77, 1, 35 - 40. View Article
Prins, M. J., Ptasinski, K. J., Janssen, F. J. J. G. (2006) Torrefaction of wood. Part 1. Weight loss kinetics. Journal of Analytical and Applied Pyrolysis 77, 1, 28 - 34. View Article
Romero, I., Moya, M., Sánchez, S., Ruiz, E., Castro, E., Bravo, V. (2007) Ethanolic fermentation of phosphoric acid hydrolysates from olive tree pruning. Industrial Crops and Products 25, 2, 160 - 168. View Article
Teymouri, F., Laureano-Perez, L., Alizadeh, H., Dale, B. E. (2005) Optimization of the ammonia fiber explosion (AFEX) treatment parameters for enzymatic hydrolysis of corn stover. Bioresource Technology 96, 18, 2014 - 2018. View Article
Várhegyi, G., Bobály, B., Jakab, E., Chen, H. (2011) Thermogravimetric study of biomass pyrolysis Kinetics. A distributed activation energy model with prediction Tests. Energy Fuels 25, 24 - 32. View Article
Wang, G., Luo, Y. H., Deng, J., Kuang, J. H., Xhang, Y. L. (2006) Pretreatment of biomass by torrefaction. Environmental Research, Chinese Science Bulletin 561442 - 1448. View Article
Williams, B. C., McMullan, J. T., McCahey, S. (2001) An initial Asessment of spent mushroom compost as a potential energy feedstock. Bioresource Technology 79, 3, 227 - 230. View Article
Wyman, C. E., Dale, B. E., Elander, R. T., Holtzapple, M., Ladisch, M. R., Lee, Y. Y. (2005) Coordinated development of leading biomass pretreatment technologies. Bioresource Technology 96, 18, 1959 - 1966. View Article
Xiang, Q., Lee, Y. Y., Torget, R. W. (2004) Kinetics of glucose decomposition during dilute-acid hydrolysis of lignocellulosic biomass. Applied Biochemistry and Biotechnology 113 - 116, 12, 1127-1138. View Article
Yat, S. C., Berger, A., Shonnar, D. R. (2008) Kinetic characterization for dilute sulfuric acid hydrolysis for timber and varieties and switchgrass. Bioresource Technology 99, 3855 - 3863. View Article
Website References
Mushroom Festival Inc. Mushroom Festival. Beacon Technologies. September 1, 2012. Accessed September 19, 2012. View Article